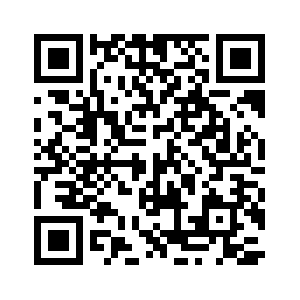
Nanorobots in Medicine: Advancing Healthcare through Molecular Engineering: A Comprehensive Review
Nanotechnology受け取った 18 Nov 2024 受け入れられた 27 Nov 2024 オンラインで公開された 28 Nov 2024
ISSN: 2995-8067 | Quick Google Scholar
Next Full Text
AI-Driven Smart Auditory Health Systems: Bridging Audiology and Public Health in Low- and Middle-Income Countries
受け取った 18 Nov 2024 受け入れられた 27 Nov 2024 オンラインで公開された 28 Nov 2024
Nanotechnology, particularly nanorobotics, has emerged as a transformative force in modern medicine. Nanorobots, designed at the molecular scale, hold promise for a range of medical applications, including targeted drug delivery, early disease diagnostics, minimally invasive surgeries, and precise infection control. Their unique ability to interact with biological systems at the cellular level opens avenues for significant advancements in treatment protocols, potentially overcoming current limitations in traditional therapies. This review delves into the development, mechanisms, and diverse medical applications of nanorobots, highlighting their structural components, energy sources, and propulsion methods. Additionally, we explore specific case studies in cancer treatment, infection control, and surgical innovations, assessing both the advancements and challenges associated with nanorobotic technologies. The goal is to present a comprehensive overview that underscores the potential of nanorobots to revolutionize patient care and set the stage for future research in this burgeoning field.
The ability to produce vast structures with essentially novel molecular organization by working at the molecular scale, atom by atom, is the very core of nanotechnology [
]. It describes the creation, manufacturing, and use of materials that fall within the nanoscale (< 1 nm - 100 nm) range. Nanoparticles' distinct physical and chemical characteristics, especially their small dimensions and large surface-to-volume ratio, enable this technology to get past obstacles and reach biological systems and molecules [ ]. Scientists anticipate gaining the benefits of nanotechnology's use in nearly every aspect since they believe it to be the science of the future [ , ]. By increasing the precision, sensitiveness, and speed of medical examinations, nanotechnology has the capability to completely transform the diagnostics industry [ ]. Many medical difficulties, including illness diagnosis, drug discovery, tailored medical procedures, cancer therapy, pharmaceutical discoveries, and the newest medical equipment and procedures, are currently improving the usage of nano-biotechnology, from diagnosis to treatment [ ]. In the field of delivery of drugs, new research approaches are growing because of the emergence of nanotechnology. Chronic intracellular infections could gain benefit from the use of drug delivery systems created with nanotechnology [ ].The field of nanorobotics, which is a subfield of nanotechnology, studies the design, programming, manufacture, and control of nanoscale robots [
]. A multidisciplinary topic that is expanding quickly is nanorobotics, which deals with the creation and application of devices the size of molecules [ ]. Fundamentally, nanorobots are nanodevices that are used to treat or protect humans from infections. The world's first nanorobot computing system for single-cell study and manipulation was developed by Professor Toshio Fukuda, who was the founding father of micro/nanorobots around the early 2000s [ ]. Carbon is the primary element utilized in nanorobots due to its strength and inertness in the form of fullerene and diamond. The additional elements, which can be used on a nanoscale, are silicon, fluorine, sulphur, nitrogen, oxygen, and hydrogen. To prevent the human immune system from attacking them, they often have an external passive diamond covering [ ]. They are created to precisely carry out specific functions or tasks on nanoscale dimensions of 1 nm - 100 nm [ ]. Target identification, medication administration, and minimally invasive surgery are some of the main medical uses for nanorobots [ , ]. It is believed that the toxicity issue caused by excessive medication usage would be resolved by determining the exact delivery ability of micro/nanorobots within the intended region [ ]. The creation and application of small robots, or nanorobots, capable of carrying out surgical operations with extreme efficiency and precision is known as surgical nanorobotics [ ].The goal of the review on nanorobots in medical applications is usually to explore the new opportunities of these tiny devices in the field of medicine. Designing and building nanorobots, understanding their processes of action, and using them for targeted medicine administration, illness diagnostics, and therapy are all part of its goal. Through tailored biosensing, the paper highlights how nanorobots might improve imaging methods and facilitate early illness identification in diagnostics. It looks at their capacity for accurate medication administration, reducing adverse effects, and optimizing therapeutic efficacy. The paper addresses the prospect of nanorobots to carry out minimally invasive surgical operations, enabling increased accuracy and quicker recovery periods. Highlighting the developments in nanotechnology, evaluating the present level of research, talking about the difficulties and constraints in using nanorobots in practice, and speculating about potential future routes for their development are the goals of the review. The review's ultimate goal is to inform and promote additional research in the area by highlighting the revolutionary possibilities of nanorobots in enhancing patient outcomes and transforming medical procedures.
Material used in designing a nanorobot: The biocompatibility of components was the main factor taken into account while designing nanorobots, which operate at nanoscale sizes inside tumor tissues and cells. The majority of materials used to make nanorobots are biocompatible or biodegradable. When their functions are completed, these biodegradable compounds can disintegrate or vanish. Nanoparticles should be able to do a variety of precise functions in the interim, such as detecting the existence of tumor cells or tissues, delivering and releasing nanocargoes in response to physical signals, identifying specific disease biomarkers, detecting changes in local pH and temperature, and more [
- ]. The nanorobot's body will be made of carbon nanotubes because of their inherent ability to absorb near-infrared light waves, which are harmless when they pass through human cells. To prevent collisions, the nanorobot's body is equipped with ultrasonic sensors. Flagella motors, a type of rotary motor with remarkable torque generation capabilities, power a nanorobot by propelling an extended, slender, and helical filament that extends multiple cellular structures into the surrounding medium. These aid in the cell's decision-making process based on changes in the proportion of vital nutrients in the environment [ ].Components of nanorobots: A nanorobot's many parts include its power source, fuel buffer tank, detectors, motors, manipulative devices, computers onboard, pumps, pressure tanks, and structural support. A nanorobot's substructures include:
Types of nanorobots:
Preparation method of micro/nanorobots: The two primary manufacturing approaches for micro/nanorobots are top-down and bottom-up. These include the roll-up process, laser direct writing 3D printing technology, and physical vapor deposition techniques (direct deposition and grazing angle deposition). Wet chemical synthesis and template electrochemical deposition technologies are part of the bottom-up approach, and they will be discussed individually below:
Micro/nanorobots may move collectively as well as alone. The driving mode will have an impact on the biocompatibility, controllability, and speed of movement of micro/nanorobots, which will impact their use in biological beings. The proactive motion of micro/nanorobots primarily depends on converting a local chemical (such as H2O2, urea, etc.) Or physical energy (such as light, ultrasound, magnetic fields, etc.) Or by microorganisms or cells (such as sperm, etc.) Toward mechanical propulsion in order to overcome the difficulties in low Reynolds fluid [
].The two driving modes for nanorobots are passive drive and active drive [
]. The driving components are what allow a nanorobot to move and operate. The former is employed for bodily entrance and nanorobot control, whereas active drive powers the nanorobot using a membrane, pumps, electric nanomotor, or onboard molecular motor [ ]. The work requires a sufficient energy source because energy is lost during movement, running, and information transmission; this runs counter to the nanorobot's size restriction. Energy may be obtained internally from microchemical cells and transformed forms like ATP motors, fuel cells, and so on. Two types of external energies are further distinguished: one uses contact to disperse energy as current or light, while the other uses an onboard converter to transform external energy [ ].Numerous functions related to diagnosing, tracking, and treating critical illnesses can be carried out by medical nanorobots. The human body has precise places and targets to which these nanorobots may deliver medications. The following are some possible uses for nanorobots: Gene therapy, drug administration, body monitoring, dentistry, surgery, cancer diagnosis and treatment, delicate procedures, diagnostics, and controlling infections [
].Micro/nanorobots have demonstrated strong capabilities in the areas of sensing detection, detoxification, and purification in recent research. Controllable driving features allow microrobots to actively hunt for things to be cleaned, resulting in a significant increase in the efficiency of detection and removal [
- ].DNA is used to create the hexagonal barrel-shaped nanorobot, which can carry a range of payloads. Two "locks" made of short strands of DNA called aptamers, which may attach to antigen targets, hold it together. The robot performs its function when the locks are unlocked in response to antigens on the outer layers of particular cells [
].By using effective chemical propulsion into the stomach fluid, magnesium (Mg)-based acidic-driven micromotors in pH-responsive nanorobots have been shown to be able to neutralize gastric acid both independently and over time [
]. When the stomach acid neutralizes the magnesium-coated ph-responsive polymer layer of these micromotors, the encapsulated payload—in this case, dye as a model payload—is released on its own. These micromotors were tested in mice and showed no discernible toxicity or disruption of stomach function. All things considered, these micromotors have the unusual twin functions of ph-responsive release of drugs and acid neutralization, making them a very attractive framework for pharmaceutical delivery to treat a range of stomach disorders. Another experiment used a mouse model to treat stomach infections caused by bacteria (Helicobacter pylori) using identical synthetic catalytic magnesium-based micromotors filled with the antibiotic clarithromycin [ ]. When the medicine was released, the magnesium within the micromotor neutralized the stomach acid locally, boosting the drug's efficacy without appearing to be harmful. Drugs might be effectively put into these magnesium-based micromotors that run on acid to demonstrate a noticeable bactericidal effect. A ph-responsive polymer-coated gold nanowire nanomotor driven by ultrasonic (US) was assessed [ ]. Before it reached the intracellular area, the encapsulated caspase-3 (a model enzyme) was shielded from release and inactivation by the nanomotors. The eudragit polymer covering was broken down by exposure to elevated intracellular pH, which released the enzyme and resulted in cell death [ ].Blood clot removal and artery cleaning: One nanobot that is intended to function as artificial platelets is called Clottocyte. Within a second, hemostasis would be fully achieved. The normal hemostatic system takes 2–5 minutes to finish the entire process, whereas the reaction time is 100–1000 times quicker [
]. Blood loss and clotting time have decreased with the use of nanobots as clottocytes. Again, it is discovered that certain individuals get unusual blood clots. The use of corticosteroids to treat this anomaly is linked to adverse consequences such as allergic reactions, lung damage, and hormone secretion. Clottocytes are an alternate therapy that doesn't have these negative effects [ ]. It is possible to identify and eliminate the plaque or blood vessel obstructing elements that cause myocardial infarction using nanobot molecules. While the traditional method of treating myocardial infarction, such as angioplasty, is dependent on surgical expertise and occasionally has adverse consequences, the procedure that nanobots follow is impervious to these [ ]. Heart bypass surgery is used to treat coronary artery disease in patients. To improve blood flow to the cardiac muscles, this is done. The procedure may bypass many arteries, however, there are certain risks associated with this approach. An alternative method of operation is to utilize a nanorobot. An electric motor is coupled to the inner region of the nanorobot, which is connected to the outside region for circulation. The electric motor is integrated with a rotating needle, a camera, a microcontroller, and an arterial thermometer. Everything will be managed by the microprocessor [ , ]. To track the motions of the nanorobot, radioactive material is injected into the outside area. With a magnetic switch, this may be changed at any moment. The induced nanorobot is capable of removing the plaque and pulverizing it into tiny bits. Upon completion of the action, it is extracted by instructing the nanorobot to attach itself to a blood artery that is readily accessible from the exterior [ ].In the early stages of cancer growth, tumor cells are detected using nanorobots equipped with chemical biosensors, or nanosensors. Malignant cells throughout the body will be detected by this nanosensor. Nanorobots can assist with such crucial parts of cancer treatment because of their ability to travel as bloodborne gadgets. Early identification of tumor cells within the patient's body can be accomplished using nanorobots equipped with chemical biosensors [
- ].Effective instruments for identifying biological dangers and removing their powerful effects are the latest microrobotic techniques.
Spores produced by certain bacteria can withstand treatment and can persist for decades in the environment. For instance, the electrodeposition approach was utilized to develop (COOH-PPy): poly polystyrene sulfonate (PEDOT)/Ni/Pt multilayer microrobots for the treatment of Bacillus anthracis (B. anthracis), a hazardous spore that causes anthrax in people and animals. Through covalent bonding, the anti-B antibody has been altered to guarantee the spore's capture by the carboxyl chains on the molecular robot's exterior. The Pt layer of the molecular robot, which uses H2O2 fuel to create O2 bubbles, supplies the propulsion mechanism, while the Ni layer serves as a magnetic guide. 90% of the nanorobots were able to drift in aqueous solutions at a speed of about 250 µm s−1 when 3% H2O2 was present, according to the research. One example of a dynamic multifunctional system that can quickly identify, isolate, detect, and destroy biological threats is this approach [
].The most frequent cause of pediatric UTIs is E. coli, which also has an irreversible impact on water quality [
]. Delezuk, et al. for instance, investigated the use of self-propelled microrobots based on alginate and chitosan to remove E. coli from tainted liquid. Magnesium (Mg) and water first interacted to initiate propulsion. The constructed molecular robots can move up to 36.5 µm/s in drinking water and 72.6 µm/s in seawater, according to their findings. In ten minutes, the molecular robot's bacterial killing effectiveness was 96%. Another experiment in San Diego County found that the eco-friendly micro-/nanorobots, which are likewise based on chitosan, were able to kill over 90% of E. coli [ ]. The magnetic helix-based physiologically interfaced platelets membrane-coated nanorobots (PL-nanorobots) have the ability to attach to bacteria and other pathogens via the human platelets' plasma membrane. One crucial characteristic of platelet-mimicking nanorobots is their resistance to biofouling, which enables them to be effectively and permanently driven in biological fluids [ ].Nanorobots must exhibit exceptional biocompatibility to minimize immune rejection and toxicity in human systems. Despite advancements in biomaterials, ensuring long-term safety remains a critical challenge. The interaction of nanorobots with complex biological environments often leads to unpredictable immune responses, necessitating further innovation in material engineering and coating technologies to achieve greater physiological compatibility.
The production of nanorobots involves intricate fabrication processes requiring high precision and scalability. Techniques such as self-assembly, microfabrication, and molecular design are resource-intensive and present challenges in reproducibility and cost-efficiency. Overcoming these barriers requires integrating advanced manufacturing methods, including 3D printing at the nanoscale and automated systems, to streamline large-scale production.
The regulatory landscape for nanomedicine is still evolving. Establishing standardized guidelines for testing, clinical trials, and commercialization is crucial. Additionally, ethical concerns surrounding the use of autonomous nanomachines in living systems demand rigorous oversight to prevent misuse and ensure patient safety.
Nanorobots signify a revolutionary leap in precision medicine, with the potential to overcome the limitations of conventional therapies. Emerging advancements in propulsion systems, energy efficiency, and sensor technologies may enable nanorobots to navigate intricate biological environments with precision. Future applications could extend to personalized medicine, where nanorobots are programmed to target patient-specific genetic and molecular profiles.
Furthermore, interdisciplinary collaborations between materials scientists, biomedical engineers, and clinical researchers are essential for addressing current limitations. Integration of artificial intelligence and machine learning in nanorobotics may also unlock innovative diagnostic and therapeutic capabilities.
The integration of nanorobotics into medical practice represents a frontier that blends engineering precision with clinical efficacy. The application of nanorobots in targeted drug delivery, diagnostics, and minimally invasive surgeries has already demonstrated encouraging results, showcasing their potential to enhance treatment specificity and minimize systemic side effects. However, several challenges, such as biocompatibility, manufacturing complexity, and regulatory hurdles, must be addressed to translate laboratory achievements into clinical reality. Continued interdisciplinary research and collaboration are essential to overcoming these obstacles. As technological innovations evolve, the horizon for nanorobots expands, promising a future where precision medicine becomes the norm, leading to better patient outcomes and more personalized healthcare solutions.
M.A. carried out all the literature searches. V.G. and M.A. carried out the writing of the manuscript. All the authors have read and approved the final version of the manuscript.
The authors are highly grateful to Gurugram Global College of Pharmacy, Gurgaon (Haryana), and Jamia Hamdard, New Delhi.
Bhushan B. Introduction to nanotechnology: history, status, and importance of nanoscience and nanotechnology education. Global Perspectives of Nanoscience and Engineering Education. 2016:1-31.
Kim BY, Rutka JT, Chan WC. Nanomedicine. N Engl J Med. 2010 Dec 16;363(25):2434-43. doi: 10.1056/NEJMra0912273. PMID: 21158659.
Misra R, Acharya S, Sahoo SK. Cancer nanotechnology: application of nanotechnology in cancer therapy. Drug Discov Today. 2010 Oct;15(19-20):842-50. doi: 10.1016/j.drudis.2010.08.006. Epub 2010 Aug 18. PMID: 20727417.
Bhushan B. Introduction to nanotechnology. Springer handbook of nanotechnology. 2017:1-9.
Fox KE, Tran NL, Nguyen TA, Nguyen TT, Tran PA. Surface modification of medical devices at nanoscale—Recent development and translational perspectives. InBiomaterials in translational medicine. Academic Press. 2019;163-189.
Malik S, Muhammad K, Waheed Y. Emerging Applications of Nanotechnology in Healthcare and Medicine. Molecules. 2023 Sep 14;28(18):6624. doi: 10.3390/molecules28186624. PMID: 37764400; PMCID: PMC10536529.
Elegbede JA, Lateef A. Green nanotechnology in Nigeria: the research landscape, challenges and prospects. Ann Sci Technol. 2019;4(2):6-38.
Ummat A, Dubey A, Mavroidis C. Bio-nanorobotics: a field inspired by nature. InBiomimetics 2005 Nov 2 (pp. 219-246). CRC Press.
Kopperger E, List J, Madhira S, Rothfischer F, Lamb DC, Simmel FC. A self-assembled nanoscale robotic arm controlled by electric fields. Science. 2018 Jan 19;359(6373):296-301. doi: 10.1126/science.aao4284. PMID: 29348232.
Fukuda T, Kajima H, Hasegawa Y. Intelligent robots as artificial living creatures. Artif Life Robotics. 2004 Dec;8:101-10.
Mazumder S, Biswas GR, Majee SB. Applications of nanorobots in medical techniques. IJPSR. 2020;11:3150
Bi C, Guix M, Johnson BV, Jing W, Cappelleri DJ. Design of Microscale Magnetic Tumbling Robots for Locomotion in Multiple Environments and Complex Terrains. Micromachines (Basel). 2018 Feb 3;9(2):68. doi: 10.3390/mi9020068. PMID: 30393344; PMCID: PMC6187462.
Rothemund PW. Folding DNA to create nanoscale shapes and patterns. Nature. 2006 Mar 16;440(7082):297-302. doi: 10.1038/nature04586. PMID: 16541064.
Kay ER, Leigh DA, Zerbetto F. Synthetic molecular motors and mechanical machines. Angew Chem Int Ed Engl. 2007;46(1-2):72-191. doi: 10.1002/anie.200504313. PMID: 17133632.
Soto F, Wang J, Ahmed R, Demirci U. Medical Micro/Nanorobots in Precision Medicine. Adv Sci (Weinh). 2020 Oct 4;7(21):2002203. doi: 10.1002/advs.202002203. PMID: 33173743; PMCID: PMC7610261.
Yoon HJ, Kim SW. Nanogenerators to power implantable medical systems. Joule. 2020;4(7):1398-407.
Chen C, Karshalev E, Li J, Soto F, Castillo R, Campos I, Mou F, Guan J, Wang J. Transient Micromotors That Disappear When No Longer Needed. ACS Nano. 2016 Nov 22;10(11):10389-10396. doi: 10.1021/acsnano.6b06256. Epub 2016 Oct 28. PMID: 27783486.
Pijpers IAB, Cao S, Llopis-Lorente A, Zhu J, Song S, Joosten RRM, Meng F, Friedrich H, Williams DS, Sánchez S, van Hest JCM, Abdelmohsen LKEA. Hybrid Biodegradable Nanomotors through Compartmentalized Synthesis. Nano Lett. 2020 Jun 10;20(6):4472-4480. doi: 10.1021/acs.nanolett.0c01268. Epub 2020 May 22. PMID: 32427492; PMCID: PMC7291354.
Wu Z , Chen Y , Mukasa D , Pak OS , Gao W . Medical micro/nanorobots in complex media. Chem Soc Rev. 2020 Nov 21;49(22):8088-8112. doi: 10.1039/d0cs00309c. Epub 2020 Jun 29. PMID: 32596700.
Guimarães CF, Gasperini L, Marques AP, Reis RL. The stiffness of living tissues and its implications for tissue engineering. Nat Rev Mater. 2020;5(5):351-70.
Senanayake A, Sirisinghe RG, Mun PS. Nanorobot: Modelling and Simulation. In: International Conference on Control, Instrumentation and Mechatronics Engineering (CIM'07); 2007 May 28; Johor Bahru, Johor, Malaysia.
Mehta J, Borkhataria C, Tejura MN. Nanorobot: A life-saving device for the pharmaceutical and medical industries. Int J Creat Res Thoughts. 2023;11(4).
Padshala R, Rajan V, Patani P. Nanobots: future and development. J Pharm Neg Results. 2022;1967-75.
Li J, Li X, Luo T, Wang R, Liu C, Chen S, Li D, Yue J, Cheng SH, Sun D. Development of a magnetic microrobot for carrying and delivering targeted cells. Sci Robot. 2018 Jun 27;3(19):eaat8829. doi: 10.1126/scirobotics.aat8829. PMID: 33141689.
Genchi GG, Marino A, Tapeinos C, Ciofani G. Smart Materials Meet Multifunctional Biomedical Devices: Current and Prospective Implications for Nanomedicine. Front Bioeng Biotechnol. 2017 Dec 18;5:80. doi: 10.3389/fbioe.2017.00080. PMID: 29326928; PMCID: PMC5741658.
Farsad N, Yilmaz HB, Eckford A, Chae CB, Guo W. A comprehensive survey of recent advancements in molecular communication. IEEE Commun Surv Tutor. 2016;18(3):1887-919.
Kumar SS, Nasim BP, Abraham E. Nanorobots: a future device for diagnosis and treatment. J Pharm Pharmaceut. 2018;5(1):44-9.
Freitas Jr RA. Microbivores: artificial mechanical phagocytes using digest and discharge protocol. J Evol Technol. 2005;14(1):54-106.
Mujtaba J, Liu J, Dey KK, Li T, Chakraborty R, Xu K, Makarov D, Barmin RA, Gorin DA, Tolstoy VP, Huang G. Micro‐bio‐chemo‐mechanical‐systems: micromotors, microfluidics, and nanozymes for biomedical applications. Adv Mater. 2021;33(22):2007465.
Mei Y, Huang G, Solovev AA, Ureña EB, Mönch I, Ding F, Reindl T, Fu RK, Chu PK, Schmidt OG. Versatile approach for integrative and functionalized tubes by strain engineering of nanomembranes on polymers. Adv Mater. 2008;20(21):4085-90.
Zhang L, Abbott JJ, Dong L, Kratochvil BE, Bell D, Nelson BJ. Artificial bacterial flagella: fabrication and magnetic control. Appl Phys Lett. 2009;94(6).
Li D , Liu Y , Yang Y , Shen Y . A fast and powerful swimming microrobot with a serrated tail enhanced propulsion interface. Nanoscale. 2018 Nov 1;10(42):19673-19677. doi: 10.1039/c8nr04907f. PMID: 30209454.
Gao W, Sattayasamitsathit S, Orozco J, Wang J. Highly efficient catalytic microengines: template electrosynthesis of polyaniline/platinum microtubes. J Am Chem Soc. 2011 Aug 10;133(31):11862-4. doi: 10.1021/ja203773g. Epub 2011 Jul 18. PMID: 21749138.
Dai B, Wang J, Xiong Z, Zhan X, Dai W, Li CC, Feng SP, Tang J. Programmable artificial phototactic microswimmer. Nat Nanotechnol. 2016 Dec;11(12):1087-1092. doi: 10.1038/nnano.2016.187. Epub 2016 Oct 17. PMID: 27749832.
Joh H, Fan DE. Materials and Schemes of Multimodal Reconfigurable Micro/Nanomachines and Robots: Review and Perspective. Adv Mater. 2021 Oct;33(39):e2101965. doi: 10.1002/adma.202101965. Epub 2021 Aug 19. PMID: 34410023.
Villa K, Viktorova J, Plutnar J, Ruml T, Hoang L, Pumera M. Chemical microrobots as self-propelled microbrushes against dental biofilm. Cell Rep Phys Sci. 2020;1(9).
Chen Y, Shi Y. Characterizing the autonomous motions of linear catalytic nanomotors using molecular dynamics simulations. J Phys Chem C. 2011;115(40):19588-97.
Gao W, Dong R, Thamphiwatana S, Li J, Gao W, Zhang L, Wang J. Artificial micromotors in the mouse's stomach: a step toward in vivo use of synthetic motors. ACS Nano. 2015 Jan 27;9(1):117-23. doi: 10.1021/nn507097k. Epub 2015 Jan 8. PMID: 25549040; PMCID: PMC4310033.
Hermanová S, Pumera M. Biocatalytic Micro- and Nanomotors. Chemistry. 2020 Sep 1;26(49):11085-11092. doi: 10.1002/chem.202001244. Epub 2020 Jul 7. PMID: 32633441.
Xu L, Mou F, Gong H, Luo M, Guan J. Light-driven micro/nanomotors: from fundamentals to applications. Chem Soc Rev. 2017 Nov 13;46(22):6905-6926. doi: 10.1039/c7cs00516d. PMID: 28949354.
Wang B, Kostarelos K, Nelson BJ, Zhang L. Trends in Micro-/Nanorobotics: Materials Development, Actuation, Localization, and System Integration for Biomedical Applications. Adv Mater. 2021 Jan;33(4):e2002047. doi: 10.1002/adma.202002047. Epub 2020 Dec 4. PMID: 33617105.
Xu H, Medina-Sánchez M, Magdanz V, Schwarz L, Hebenstreit F, Schmidt OG. Sperm-Hybrid Micromotor for Targeted Drug Delivery. ACS Nano. 2018 Jan 23;12(1):327-337. doi: 10.1021/acsnano.7b06398. Epub 2017 Dec 13. PMID: 29202221.
Alapan Y, Yasa O, Schauer O, Giltinan J, Tabak AF, Sourjik V, Sitti M. Soft erythrocyte-based bacterial microswimmers for cargo delivery. Sci Robot. 2018 Apr 25;3(17):eaar4423. doi: 10.1126/scirobotics.aar4423. PMID: 33141741.
Jiang HW, Wang SG, Xu W, Zhang ZZ, He L. Construction of medical nanorobot. In: 2005 IEEE International Conference on Robotics and Biomimetics (ROBIO); IEEE. 2005;151-154. x
Giri G, Maddahi Y, Zareinia K. A brief review on challenges in design and development of nanorobots for medical applications. Appl Sci. 2021;11(21):10385.
Freitas Jr RA. Medical nanorobotics: the long-term goal for nanomedicine. In: Nanomedicine: design of particles, sensors, motors, implants, robots, and devices. Norwood, MA: Artech House; 2009;367-92.
Zhang Y, Zhang L, Yang L, Vong CI, Chan KF, Wu WKK, Kwong TNY, Lo NWS, Ip M, Wong SH, Sung JJY, Chiu PWY, Zhang L. Real-time tracking of fluorescent magnetic spore-based microrobots for remote detection of difftoxins. Sci Adv. 2019 Jan 11;5(1):eaau9650. doi: 10.1126/sciadv.aau9650. PMID: 30746470; PMCID: PMC6357761.
Wang Q, Li T, Fang D, Li X, Fang L, Wang X, Mao C, Wang F, Wan M. Micromotor for removal/detection of blood copper ion. Microchem J. 2020;158:105125.
Molinero-Fernández Á, Moreno-Guzmán M, Arruza L, López MÁ, Escarpa A. Polymer-Based Micromotor Fluorescence Immunoassay for On-the-MoveSensitive Procalcitonin Determination in Very Low Birth Weight Infants' Plasma. ACS Sens. 2020 May 22;5(5):1336-1344. doi: 10.1021/acssensors.9b02515. Epub 2020 May 5. PMID: 32204587.
Cavalcanti A. Assembly automation with evolutionary nanorobots and sensor-based control applied to nanomedicine. IEEE Trans Nanotechnol. 2003;2(2):82-7.
Murphy D, Challacombe B, Khan MS, Dasgupta P. Robotic technology in urology. Postgrad Med J. 2006 Nov;82(973):743-7. doi: 10.1136/pgmj.2006.048140. PMID: 17099094; PMCID: PMC2660512.
Cavalcanti A, Freitas RA Jr. Nanorobotics control design: a collective behavior approach for medicine. IEEE Trans Nanobioscience. 2005 Jun;4(2):133-40. doi: 10.1109/tnb.2005.850469. PMID: 16117021.
Hogg T, Kuekes PJ. Mobile microscopic sensors for high resolution in vivo diagnostics. Nanomedicine. 2006 Dec;2(4):239-47. doi: 10.1016/j.nano.2006.10.004. PMID: 17292149.
Peng J, Freitas RA, Merkle RC. Theoretical analysis of diamond mechanosynthesis. Part I. Stability of C2 mediated growth of nanocrystalline diamond C (110) surface. J Comput Theor Nanosci. 2004;1(1):62-70.
Freitas Jr RA. Meeting the challenge of building diamondoid medical nanorobots. Int J Robotics Res. 2009 Apr;28(4):548-57.
Braun-Sand SB, Wiest O. Theoretical studies of mixed-valence transition metal complexes for molecular computing. J Phys Chem A. 2003;107(2):285-91.
Sharma G, Badescu M, Dubey A, Mavroidis C, Tomassone SM, Yarmush ML. Kinematics and workspace analysis of protein based nano-actuators. J Mech Des. 2005;127:718-727.
Makaliwe JH, Requicha AA. Automatic planning of nanoparticle assembly tasks. In: Proceedings of the 2001 IEEE International Symposium on Assembly and Task Planning (ISATP2001). IEEE. 2001;288-293.
Freitas RA Jr. Pharmacytes: an ideal vehicle for targeted drug delivery. J Nanosci Nanotechnol. 2006 Sep-Oct;6(9-10):2769-75. doi: 10.1166/jnn.2006.413. PMID: 17048481.
Chan T, inventor. Multithreaded, mixed hardware description languages logic simulation on engineering workstations. United States patent US 6,466,898. 2002 Oct 15.
Cavalcanti A, Shirinzadeh B, Freitas RA, Hogg T. Nanorobot architecture for medical target identification. Nanotechnology. 2007;19(1):015103.
Hamdi M, Ferreira A, Sharma G, Mavroidis C. Prototyping bio-nanorobots using molecular dynamics simulation and virtual reality. Microelectronics Journal. 2008;39(2):190-201.
Curtis AS, Dalby M, Gadegaard N. Cell signaling arising from nanotopography: implications for nanomedical devices. Nanomedicine (Lond). 2006 Jun;1(1):67-72. doi: 10.2217/17435889.1.1.67. PMID: 17716210.
Kubik T, Bogunia-Kubik K, Sugisaka M. Nanotechnology on duty in medical applications. Curr Pharm Biotechnol. 2005 Feb;6(1):17-33. doi: 10.2174/1389201053167248. PMID: 15727553.
Suri SS, Fenniri H, Singh B. Nanotechnology-based drug delivery systems. J Occup Med Toxicol. 2007 Dec 1;2:16. doi: 10.1186/1745-6673-2-16. PMID: 18053152; PMCID: PMC2222591.
Li D, Dong D, Lam W, Xing L, Wei T, Sun D. Automated In Vivo Navigation of Magnetic-Driven Microrobots Using OCT Imaging Feedback. IEEE Trans Biomed Eng. 2020 Aug;67(8):2349-2358. doi: 10.1109/TBME.2019.2960530. Epub 2019 Dec 18. PMID: 31869776.
Navab N, Bascle B, Loser M, Geiger B, Taylor R. Visual servoing for automatic and uncalibrated needle placement for percutaneous procedures. In: Proceedings IEEE Conference on Computer Vision and Pattern Recognition (CVPR); IEEE. 2000; 2:327-334.
Flückiger M, Neild A, Nelson BJ. Optimization of receiver arrangements for passive emitter localization methods. Ultrasonics. 2012 Mar 1;52(3):447-55.
Yan X, Zhou Q, Vincent M, Deng Y, Yu J, Xu J, Xu T, Tang T, Bian L, Wang YJ, Kostarelos K, Zhang L. Multifunctional biohybrid magnetite microrobots for imaging-guided therapy. Sci Robot. 2017 Nov 22;2(12):eaaq1155. doi: 10.1126/scirobotics.aaq1155. PMID: 33157904.
Ikemoto Y, Ito K, Sato K. Another Mechanism for Gait Generation: Mechanical Stabilization Can Spontaneously Realize Walking in a Two-Legged Robot.
Cho EC, Glaus C, Chen J, Welch MJ, Xia Y. Inorganic nanoparticle-based contrast agents for molecular imaging. Trends Mol Med. 2010 Dec;16(12):561-73. doi: 10.1016/j.molmed.2010.09.004. Epub 2010 Nov 10. PMID: 21074494; PMCID: PMC3052982.
Lee S, Chan Kwon I, Kim K. Multifunctional nanoparticles for cancer theragnosis. In: Nanoplatform-Based Molecular Imaging. 2011;541-63.
Kievit FM, Zhang M. Surface engineering of iron oxide nanoparticles for targeted cancer therapy. Acc Chem Res. 2011 Oct 18;44(10):853-62. doi: 10.1021/ar2000277. Epub 2011 Apr 29. PMID: 21528865; PMCID: PMC3192288.
Lee H, Shin TH, Cheon J, Weissleder R. Recent Developments in Magnetic Diagnostic Systems. Chem Rev. 2015 Oct 14;115(19):10690-724. doi: 10.1021/cr500698d. Epub 2015 Aug 10. PMID: 26258867; PMCID: PMC5791529.
Petrovic N, Petrovic MJ, Sreckovic S, Jovanovic S, Todorovic D, Vulovic TS. Nanotechnology in ophthalmology. Commercialization of Nanotechnologies–A Case Study Approach. 2018:275-97.
Johnston AP, Caruso F. Stabilization of DNA multilayer films through oligonucleotide crosslinking. Small. 2008 May;4(5):612-8. doi: 10.1002/smll.200700813. PMID: 18393261.
Guyer RA, Macara IG. Loss of the polarity protein PAR3 activates STAT3 signaling via an atypical protein kinase C (aPKC)/NF-κB/interleukin-6 (IL-6) axis in mouse mammary cells. J Biol Chem. 2015 Mar 27;290(13):8457-68. doi: 10.1074/jbc.M114.621011. Epub 2015 Feb 5. PMID: 25657002; PMCID: PMC4375497.
Dutta D, Sailapu SK. Biomedical applications of nanobots. In: Intelligent Nanomaterials for Drug Delivery Applications. Elsevier. 2020; 179-95.
Freitas RA. Nanotechnology, nanomedicine and nanosurgery. Int J Surg. 2005;3(4):243-6. doi: 10.1016/j.ijsu.2005.10.007. Epub 2005 Nov 28. PMID: 17462292.
Sivasankar M, Durairaj R. Brief review on nano robots in bio medical applications. Adv Robot Autom. 2012;1(101):2.
Rifat T, Hossain MS, Alam MM, Rouf AS. A review on applications of nanobots in combating complex diseases. Bangladesh Pharm J. 2019;22(1):99-108.
Li J, Esteban-Fernández de Ávila B, Gao W, Zhang L, Wang J. Micro/Nanorobots for Biomedicine: Delivery, Surgery, Sensing, and Detoxification. Sci Robot. 2017 Mar 15;2(4):eaam6431. doi: 10.1126/scirobotics.aam6431. Epub 2017 Mar 1. PMID: 31552379; PMCID: PMC6759331.
Wu Z, Li J, de Ávila BE, Li T, Gao W, He Q, Zhang L, Wang J. Water-powered cell-mimicking Janus micromotor. Adv Funct Mater. 2015;25(48):7497-501.
Wu Z, Wu Y, He W, Lin X, Sun J, He Q. Self-propelled polymer-based multilayer nanorockets for transportation and drug release. Angew Chem Int Ed Engl. 2013 Jul 1;52(27):7000-3. doi: 10.1002/anie.201301643. Epub 2013 May 23. PMID: 23703837.
Qiu F, Fujita S, Mhanna R, Zhang L, Simona BR, Nelson BJ. Magnetic helical microswimmers functionalized with lipoplexes for targeted gene delivery. Adv Funct Mater. 2015;25(11):1666-71.
Bozuyuk U, Yasa O, Yasa IC, Ceylan H, Kizilel S, Sitti M. Light-Triggered Drug Release from 3D-Printed Magnetic Chitosan Microswimmers. ACS Nano. 2018 Sep 25;12(9):9617-9625. doi: 10.1021/acsnano.8b05997. Epub 2018 Sep 11. PMID: 30203963.
Li J, Angsantikul P, Liu W, Esteban-Fernández de Ávila B, Thamphiwatana S, Xu M, Sandraz E, Wang X, Delezuk J, Gao W, Zhang L, Wang J. Micromotors Spontaneously Neutralize Gastric Acid for pH-Responsive Payload Release. Angew Chem Int Ed Engl. 2017 Feb 13;56(8):2156-2161. doi: 10.1002/anie.201611774. Epub 2017 Jan 20. PMID: 28105785; PMCID: PMC5511515.
de Ávila BE, Angsantikul P, Li J, Angel Lopez-Ramirez M, Ramírez-Herrera DE, Thamphiwatana S, Chen C, Delezuk J, Samakapiruk R, Ramez V, Obonyo M, Zhang L, Wang J. Micromotor-enabled active drug delivery for in vivo treatment of stomach infection. Nat Commun. 2017 Aug 16;8(1):272. doi: 10.1038/s41467-017-00309-w. Erratum in: Nat Commun. 2017 Oct 31;8(1):1299. doi: 10.1038/s41467-017-01616-y. PMID: 28814725; PMCID: PMC5559609.
Esteban-Fernández de Ávila B, Ramírez-Herrera DE, Campuzano S, Angsantikul P, Zhang L, Wang J. Nanomotor-Enabled pH-Responsive Intracellular Delivery of Caspase-3: Toward Rapid Cell Apoptosis. ACS Nano. 2017 Jun 27;11(6):5367-5374. doi: 10.1021/acsnano.7b01926. Epub 2017 May 5. PMID: 28467853; PMCID: PMC5894870.
Liu D, Yang F, Xiong F, Gu N. The Smart Drug Delivery System and Its Clinical Potential. Theranostics. 2016 Jun 7;6(9):1306-23. doi: 10.7150/thno.14858. PMID: 27375781; PMCID: PMC4924501.
Singh AV, Ansari MHD, Laux P, Luch A. Micro-nanorobots: important considerations when developing novel drug delivery platforms. Expert Opin Drug Deliv. 2019 Nov;16(11):1259-1275. doi: 10.1080/17425247.2019.1676228. Epub 2019 Oct 14. PMID: 31580731.
Chen C, Chen L, Wang P, Wu LF, Song T. Steering of magnetotactic bacterial microrobots by focusing magnetic field for targeted pathogen killing. J Magn Magn Mater. 2019;479:74-83.
Lin Z, Gao C, Wang D, He Q. Bubble-Propelled Janus Gallium/Zinc Micromotors for the Active Treatment of Bacterial Infections. Angew Chem Int Ed Engl. 2021 Apr 12;60(16):8750-8754. doi: 10.1002/anie.202016260. Epub 2021 Mar 9. PMID: 33481280.
Farahani A, Farahani A. An adaptive controller for motion control of nanorobots inside human blood vessels. Biosci Biotechnol Res Commun. 2016 Jul 1;9(3):546-52.
Manjunath A, Kishore V. The promising future in medicine: nanorobots. Biomed Sci Eng. 2014;2(2):42-7.
Biswas O, Sen A. Nanorobot the expected ever reliable future asset in diagnosis, treatment and therapy. In: Foundations and Frontiers in Computer, Communication and Electrical Engineering: Proceedings of the 3rd International Conference C2E2; 2016; 451.
Belachew GT. Disease fighting machines inside the body—A review. Biomed J Sci Tech Res. 2023;49(2):40454-62.
Kwan JJ, Myers R, Coviello CM, Graham SM, Shah AR, Stride E, Carlisle RC, Coussios CC. Ultrasound-Propelled Nanocups for Drug Delivery. Small. 2015 Oct 21;11(39):5305-14. doi: 10.1002/smll.201501322. Epub 2015 Aug 21. PMID: 26296985; PMCID: PMC4660885.
Pokki J, Ergeneman O, Chatzipirpiridis G, Lühmann T, Sort J, Pellicer E, Pot SA, Spiess BM, Pané S, Nelson BJ. Protective coatings for intraocular wirelessly controlled microrobots for implantation: Corrosion, cell culture, and in vivo animal tests. J Biomed Mater Res B Appl Biomater. 2017 May;105(4):836-845. doi: 10.1002/jbm.b.33618. Epub 2016 Jan 24. PMID: 26804771.
Tripathi PA, Singh AD. Natural resources from plants in the treatment of cancer: an update. Asian J Pharm Clin Res. 2017;10(7):13-22.
Preethi R, Padma PR. Anticancer activity of silver nanobioconjugates synthesized from Piper betle leaves extract and its active compound eugenol. Int J Pharm Pharm Sci. 2016;8(9):201-5.
Safdar MH, Hussain Z, Abourehab MAS, Hasan H, Afzal S, Thu HE. New developments and clinical transition of hyaluronic acid-based nanotherapeutics for treatment of cancer: reversing multidrug resistance, tumour-specific targetability and improved anticancer efficacy. Artif Cells Nanomed Biotechnol. 2018 Dec;46(8):1967-1980. doi: 10.1080/21691401.2017.1397001. Epub 2017 Oct 30. PMID: 29082766.
da Silva Luz GV, Barros KV, de Araújo FV, da Silva GB, da Silva PA, Condori RC, Mattos L. Nanorobotics in drug delivery systems for treatment of cancer: a review. J Mat Sci Eng A. 2016;6:167-80.
Yu J, Yang C, Li J, Ding Y, Zhang L, Yousaf MZ, Lin J, Pang R, Wei L, Xu L, Sheng F, Li C, Li G, Zhao L, Hou Y. Multifunctional Fe5 C2 nanoparticles: a targeted theranostic platform for magnetic resonance imaging and photoacoustic tomography-guided photothermal therapy. Adv Mater. 2014 Jun 25;26(24):4114-20. doi: 10.1002/adma.201305811. Epub 2014 Mar 26. PMID: 24677251.
Yu J , Chen F , Gao W , Ju Y , Chu X , Che S , Sheng F , Hou Y . Iron carbide nanoparticles: an innovative nanoplatform for biomedical applications. Nanoscale Horiz. 2017 Mar 1;2(2):81-88. doi: 10.1039/c6nh00173d. Epub 2016 Dec 1. PMID: 32260669.
Sun Z, Wang T, Wang J, Xu J, Shen T, Zhang T, Zhang B, Gao S, Zhao C, Yang M, Sheng F, Yu J, Hou Y. Self-Propelled Janus Nanocatalytic Robots Guided by Magnetic Resonance Imaging for Enhanced Tumor Penetration and Therapy. J Am Chem Soc. 2023 May 24;145(20):11019-11032. doi: 10.1021/jacs.2c12219. Epub 2023 May 16. PMID: 37190936.
Choi H, Lee GH, Kim KS, Hahn SK. Light-Guided Nanomotor Systems for Autonomous Photothermal Cancer Therapy. ACS Appl Mater Interfaces. 2018 Jan 24;10(3):2338-2346. doi: 10.1021/acsami.7b16595. Epub 2018 Jan 12. PMID: 29280612.
Orozco J, Pan G, Sattayasamitsathit S, Galarnyk M, Wang J. Micromotors to capture and destroy anthrax simulant spores. Analyst. 2015 Mar 7;140(5):1421-7. doi: 10.1039/c4an02169j. Epub 2015 Jan 27. PMID: 25622851.
Mellata M. Human and avian extraintestinal pathogenic Escherichia coli: infections, zoonotic risks, and antibiotic resistance trends. Foodborne Pathog Dis. 2013 Nov;10(11):916-32. doi: 10.1089/fpd.2013.1533. Epub 2013 Aug 20. PMID: 23962019; PMCID: PMC3865812.
Delezuk JA, Ramírez-Herrera DE, Esteban-Fernández de Ávila B, Wang J. Chitosan-based water-propelled micromotors with strong antibacterial activity. Nanoscale. 2017 Feb 9;9(6):2195-2200. doi: 10.1039/c6nr09799e. PMID: 28134392.
Li J, Angsantikul P, Liu W, Esteban-Fernández de Ávila B, Chang X, Sandraz E, Liang Y, Zhu S, Zhang Y, Chen C, Gao W, Zhang L, Wang J. Biomimetic Platelet-Camouflaged Nanorobots for Binding and Isolation of Biological Threats. Adv Mater. 2018 Jan;30(2). doi: 10.1002/adma.201704800. Epub 2017 Nov 28. PMID: 29193346.
Antil M, Gupta V. Nanorobots in Medicine: Advancing Healthcare through Molecular Engineering: A Comprehensive Review. IgMin Res. November 28, 2024; 2(11): 938-949. IgMin ID: igmin271; DOI:10.61927/igmin271; Available at: igmin.link/p271
次のリンクを共有した人は、このコンテンツを読むことができます:
1Gurugram Global College of Pharmacy, Kheda Khurampur, Farrukhnagar-Haily Mandi Road, Gurgaon (Haryana), 122506, India
2School of Pharmaceutical Education and Research (SPER), Jamia Hamdard University, New Delhi, 110062, India
Address Correspondence:
Vaibhav Gupta, School of Pharmaceutical Education and Research (SPER), Jamia Hamdard University, New Delhi, 110062, India, Email: [email protected]
How to cite this article:
Antil M, Gupta V. Nanorobots in Medicine: Advancing Healthcare through Molecular Engineering: A Comprehensive Review. IgMin Res. November 28, 2024; 2(11): 938-949. IgMin ID: igmin271; DOI:10.61927/igmin271; Available at: igmin.link/p271
Copyright: © 2024 Antil M, et al. This is an open access article distributed under the Creative Commons Attribution License, which permits unrestricted use, distribution, and reproduction in any medium, provided the original work is properly cited.
Bhushan B. Introduction to nanotechnology: history, status, and importance of nanoscience and nanotechnology education. Global Perspectives of Nanoscience and Engineering Education. 2016:1-31.
Kim BY, Rutka JT, Chan WC. Nanomedicine. N Engl J Med. 2010 Dec 16;363(25):2434-43. doi: 10.1056/NEJMra0912273. PMID: 21158659.
Misra R, Acharya S, Sahoo SK. Cancer nanotechnology: application of nanotechnology in cancer therapy. Drug Discov Today. 2010 Oct;15(19-20):842-50. doi: 10.1016/j.drudis.2010.08.006. Epub 2010 Aug 18. PMID: 20727417.
Bhushan B. Introduction to nanotechnology. Springer handbook of nanotechnology. 2017:1-9.
Fox KE, Tran NL, Nguyen TA, Nguyen TT, Tran PA. Surface modification of medical devices at nanoscale—Recent development and translational perspectives. InBiomaterials in translational medicine. Academic Press. 2019;163-189.
Malik S, Muhammad K, Waheed Y. Emerging Applications of Nanotechnology in Healthcare and Medicine. Molecules. 2023 Sep 14;28(18):6624. doi: 10.3390/molecules28186624. PMID: 37764400; PMCID: PMC10536529.
Elegbede JA, Lateef A. Green nanotechnology in Nigeria: the research landscape, challenges and prospects. Ann Sci Technol. 2019;4(2):6-38.
Ummat A, Dubey A, Mavroidis C. Bio-nanorobotics: a field inspired by nature. InBiomimetics 2005 Nov 2 (pp. 219-246). CRC Press.
Kopperger E, List J, Madhira S, Rothfischer F, Lamb DC, Simmel FC. A self-assembled nanoscale robotic arm controlled by electric fields. Science. 2018 Jan 19;359(6373):296-301. doi: 10.1126/science.aao4284. PMID: 29348232.
Fukuda T, Kajima H, Hasegawa Y. Intelligent robots as artificial living creatures. Artif Life Robotics. 2004 Dec;8:101-10.
Mazumder S, Biswas GR, Majee SB. Applications of nanorobots in medical techniques. IJPSR. 2020;11:3150
Bi C, Guix M, Johnson BV, Jing W, Cappelleri DJ. Design of Microscale Magnetic Tumbling Robots for Locomotion in Multiple Environments and Complex Terrains. Micromachines (Basel). 2018 Feb 3;9(2):68. doi: 10.3390/mi9020068. PMID: 30393344; PMCID: PMC6187462.
Rothemund PW. Folding DNA to create nanoscale shapes and patterns. Nature. 2006 Mar 16;440(7082):297-302. doi: 10.1038/nature04586. PMID: 16541064.
Kay ER, Leigh DA, Zerbetto F. Synthetic molecular motors and mechanical machines. Angew Chem Int Ed Engl. 2007;46(1-2):72-191. doi: 10.1002/anie.200504313. PMID: 17133632.
Soto F, Wang J, Ahmed R, Demirci U. Medical Micro/Nanorobots in Precision Medicine. Adv Sci (Weinh). 2020 Oct 4;7(21):2002203. doi: 10.1002/advs.202002203. PMID: 33173743; PMCID: PMC7610261.
Yoon HJ, Kim SW. Nanogenerators to power implantable medical systems. Joule. 2020;4(7):1398-407.
Chen C, Karshalev E, Li J, Soto F, Castillo R, Campos I, Mou F, Guan J, Wang J. Transient Micromotors That Disappear When No Longer Needed. ACS Nano. 2016 Nov 22;10(11):10389-10396. doi: 10.1021/acsnano.6b06256. Epub 2016 Oct 28. PMID: 27783486.
Pijpers IAB, Cao S, Llopis-Lorente A, Zhu J, Song S, Joosten RRM, Meng F, Friedrich H, Williams DS, Sánchez S, van Hest JCM, Abdelmohsen LKEA. Hybrid Biodegradable Nanomotors through Compartmentalized Synthesis. Nano Lett. 2020 Jun 10;20(6):4472-4480. doi: 10.1021/acs.nanolett.0c01268. Epub 2020 May 22. PMID: 32427492; PMCID: PMC7291354.
Wu Z , Chen Y , Mukasa D , Pak OS , Gao W . Medical micro/nanorobots in complex media. Chem Soc Rev. 2020 Nov 21;49(22):8088-8112. doi: 10.1039/d0cs00309c. Epub 2020 Jun 29. PMID: 32596700.
Guimarães CF, Gasperini L, Marques AP, Reis RL. The stiffness of living tissues and its implications for tissue engineering. Nat Rev Mater. 2020;5(5):351-70.
Senanayake A, Sirisinghe RG, Mun PS. Nanorobot: Modelling and Simulation. In: International Conference on Control, Instrumentation and Mechatronics Engineering (CIM'07); 2007 May 28; Johor Bahru, Johor, Malaysia.
Mehta J, Borkhataria C, Tejura MN. Nanorobot: A life-saving device for the pharmaceutical and medical industries. Int J Creat Res Thoughts. 2023;11(4).
Padshala R, Rajan V, Patani P. Nanobots: future and development. J Pharm Neg Results. 2022;1967-75.
Li J, Li X, Luo T, Wang R, Liu C, Chen S, Li D, Yue J, Cheng SH, Sun D. Development of a magnetic microrobot for carrying and delivering targeted cells. Sci Robot. 2018 Jun 27;3(19):eaat8829. doi: 10.1126/scirobotics.aat8829. PMID: 33141689.
Genchi GG, Marino A, Tapeinos C, Ciofani G. Smart Materials Meet Multifunctional Biomedical Devices: Current and Prospective Implications for Nanomedicine. Front Bioeng Biotechnol. 2017 Dec 18;5:80. doi: 10.3389/fbioe.2017.00080. PMID: 29326928; PMCID: PMC5741658.
Farsad N, Yilmaz HB, Eckford A, Chae CB, Guo W. A comprehensive survey of recent advancements in molecular communication. IEEE Commun Surv Tutor. 2016;18(3):1887-919.
Kumar SS, Nasim BP, Abraham E. Nanorobots: a future device for diagnosis and treatment. J Pharm Pharmaceut. 2018;5(1):44-9.
Freitas Jr RA. Microbivores: artificial mechanical phagocytes using digest and discharge protocol. J Evol Technol. 2005;14(1):54-106.
Mujtaba J, Liu J, Dey KK, Li T, Chakraborty R, Xu K, Makarov D, Barmin RA, Gorin DA, Tolstoy VP, Huang G. Micro‐bio‐chemo‐mechanical‐systems: micromotors, microfluidics, and nanozymes for biomedical applications. Adv Mater. 2021;33(22):2007465.
Mei Y, Huang G, Solovev AA, Ureña EB, Mönch I, Ding F, Reindl T, Fu RK, Chu PK, Schmidt OG. Versatile approach for integrative and functionalized tubes by strain engineering of nanomembranes on polymers. Adv Mater. 2008;20(21):4085-90.
Zhang L, Abbott JJ, Dong L, Kratochvil BE, Bell D, Nelson BJ. Artificial bacterial flagella: fabrication and magnetic control. Appl Phys Lett. 2009;94(6).
Li D , Liu Y , Yang Y , Shen Y . A fast and powerful swimming microrobot with a serrated tail enhanced propulsion interface. Nanoscale. 2018 Nov 1;10(42):19673-19677. doi: 10.1039/c8nr04907f. PMID: 30209454.
Gao W, Sattayasamitsathit S, Orozco J, Wang J. Highly efficient catalytic microengines: template electrosynthesis of polyaniline/platinum microtubes. J Am Chem Soc. 2011 Aug 10;133(31):11862-4. doi: 10.1021/ja203773g. Epub 2011 Jul 18. PMID: 21749138.
Dai B, Wang J, Xiong Z, Zhan X, Dai W, Li CC, Feng SP, Tang J. Programmable artificial phototactic microswimmer. Nat Nanotechnol. 2016 Dec;11(12):1087-1092. doi: 10.1038/nnano.2016.187. Epub 2016 Oct 17. PMID: 27749832.
Joh H, Fan DE. Materials and Schemes of Multimodal Reconfigurable Micro/Nanomachines and Robots: Review and Perspective. Adv Mater. 2021 Oct;33(39):e2101965. doi: 10.1002/adma.202101965. Epub 2021 Aug 19. PMID: 34410023.
Villa K, Viktorova J, Plutnar J, Ruml T, Hoang L, Pumera M. Chemical microrobots as self-propelled microbrushes against dental biofilm. Cell Rep Phys Sci. 2020;1(9).
Chen Y, Shi Y. Characterizing the autonomous motions of linear catalytic nanomotors using molecular dynamics simulations. J Phys Chem C. 2011;115(40):19588-97.
Gao W, Dong R, Thamphiwatana S, Li J, Gao W, Zhang L, Wang J. Artificial micromotors in the mouse's stomach: a step toward in vivo use of synthetic motors. ACS Nano. 2015 Jan 27;9(1):117-23. doi: 10.1021/nn507097k. Epub 2015 Jan 8. PMID: 25549040; PMCID: PMC4310033.
Hermanová S, Pumera M. Biocatalytic Micro- and Nanomotors. Chemistry. 2020 Sep 1;26(49):11085-11092. doi: 10.1002/chem.202001244. Epub 2020 Jul 7. PMID: 32633441.
Xu L, Mou F, Gong H, Luo M, Guan J. Light-driven micro/nanomotors: from fundamentals to applications. Chem Soc Rev. 2017 Nov 13;46(22):6905-6926. doi: 10.1039/c7cs00516d. PMID: 28949354.
Wang B, Kostarelos K, Nelson BJ, Zhang L. Trends in Micro-/Nanorobotics: Materials Development, Actuation, Localization, and System Integration for Biomedical Applications. Adv Mater. 2021 Jan;33(4):e2002047. doi: 10.1002/adma.202002047. Epub 2020 Dec 4. PMID: 33617105.
Xu H, Medina-Sánchez M, Magdanz V, Schwarz L, Hebenstreit F, Schmidt OG. Sperm-Hybrid Micromotor for Targeted Drug Delivery. ACS Nano. 2018 Jan 23;12(1):327-337. doi: 10.1021/acsnano.7b06398. Epub 2017 Dec 13. PMID: 29202221.
Alapan Y, Yasa O, Schauer O, Giltinan J, Tabak AF, Sourjik V, Sitti M. Soft erythrocyte-based bacterial microswimmers for cargo delivery. Sci Robot. 2018 Apr 25;3(17):eaar4423. doi: 10.1126/scirobotics.aar4423. PMID: 33141741.
Jiang HW, Wang SG, Xu W, Zhang ZZ, He L. Construction of medical nanorobot. In: 2005 IEEE International Conference on Robotics and Biomimetics (ROBIO); IEEE. 2005;151-154. x
Giri G, Maddahi Y, Zareinia K. A brief review on challenges in design and development of nanorobots for medical applications. Appl Sci. 2021;11(21):10385.
Freitas Jr RA. Medical nanorobotics: the long-term goal for nanomedicine. In: Nanomedicine: design of particles, sensors, motors, implants, robots, and devices. Norwood, MA: Artech House; 2009;367-92.
Zhang Y, Zhang L, Yang L, Vong CI, Chan KF, Wu WKK, Kwong TNY, Lo NWS, Ip M, Wong SH, Sung JJY, Chiu PWY, Zhang L. Real-time tracking of fluorescent magnetic spore-based microrobots for remote detection of difftoxins. Sci Adv. 2019 Jan 11;5(1):eaau9650. doi: 10.1126/sciadv.aau9650. PMID: 30746470; PMCID: PMC6357761.
Wang Q, Li T, Fang D, Li X, Fang L, Wang X, Mao C, Wang F, Wan M. Micromotor for removal/detection of blood copper ion. Microchem J. 2020;158:105125.
Molinero-Fernández Á, Moreno-Guzmán M, Arruza L, López MÁ, Escarpa A. Polymer-Based Micromotor Fluorescence Immunoassay for On-the-MoveSensitive Procalcitonin Determination in Very Low Birth Weight Infants' Plasma. ACS Sens. 2020 May 22;5(5):1336-1344. doi: 10.1021/acssensors.9b02515. Epub 2020 May 5. PMID: 32204587.
Cavalcanti A. Assembly automation with evolutionary nanorobots and sensor-based control applied to nanomedicine. IEEE Trans Nanotechnol. 2003;2(2):82-7.
Murphy D, Challacombe B, Khan MS, Dasgupta P. Robotic technology in urology. Postgrad Med J. 2006 Nov;82(973):743-7. doi: 10.1136/pgmj.2006.048140. PMID: 17099094; PMCID: PMC2660512.
Cavalcanti A, Freitas RA Jr. Nanorobotics control design: a collective behavior approach for medicine. IEEE Trans Nanobioscience. 2005 Jun;4(2):133-40. doi: 10.1109/tnb.2005.850469. PMID: 16117021.
Hogg T, Kuekes PJ. Mobile microscopic sensors for high resolution in vivo diagnostics. Nanomedicine. 2006 Dec;2(4):239-47. doi: 10.1016/j.nano.2006.10.004. PMID: 17292149.
Peng J, Freitas RA, Merkle RC. Theoretical analysis of diamond mechanosynthesis. Part I. Stability of C2 mediated growth of nanocrystalline diamond C (110) surface. J Comput Theor Nanosci. 2004;1(1):62-70.
Freitas Jr RA. Meeting the challenge of building diamondoid medical nanorobots. Int J Robotics Res. 2009 Apr;28(4):548-57.
Braun-Sand SB, Wiest O. Theoretical studies of mixed-valence transition metal complexes for molecular computing. J Phys Chem A. 2003;107(2):285-91.
Sharma G, Badescu M, Dubey A, Mavroidis C, Tomassone SM, Yarmush ML. Kinematics and workspace analysis of protein based nano-actuators. J Mech Des. 2005;127:718-727.
Makaliwe JH, Requicha AA. Automatic planning of nanoparticle assembly tasks. In: Proceedings of the 2001 IEEE International Symposium on Assembly and Task Planning (ISATP2001). IEEE. 2001;288-293.
Freitas RA Jr. Pharmacytes: an ideal vehicle for targeted drug delivery. J Nanosci Nanotechnol. 2006 Sep-Oct;6(9-10):2769-75. doi: 10.1166/jnn.2006.413. PMID: 17048481.
Chan T, inventor. Multithreaded, mixed hardware description languages logic simulation on engineering workstations. United States patent US 6,466,898. 2002 Oct 15.
Cavalcanti A, Shirinzadeh B, Freitas RA, Hogg T. Nanorobot architecture for medical target identification. Nanotechnology. 2007;19(1):015103.
Hamdi M, Ferreira A, Sharma G, Mavroidis C. Prototyping bio-nanorobots using molecular dynamics simulation and virtual reality. Microelectronics Journal. 2008;39(2):190-201.
Curtis AS, Dalby M, Gadegaard N. Cell signaling arising from nanotopography: implications for nanomedical devices. Nanomedicine (Lond). 2006 Jun;1(1):67-72. doi: 10.2217/17435889.1.1.67. PMID: 17716210.
Kubik T, Bogunia-Kubik K, Sugisaka M. Nanotechnology on duty in medical applications. Curr Pharm Biotechnol. 2005 Feb;6(1):17-33. doi: 10.2174/1389201053167248. PMID: 15727553.
Suri SS, Fenniri H, Singh B. Nanotechnology-based drug delivery systems. J Occup Med Toxicol. 2007 Dec 1;2:16. doi: 10.1186/1745-6673-2-16. PMID: 18053152; PMCID: PMC2222591.
Li D, Dong D, Lam W, Xing L, Wei T, Sun D. Automated In Vivo Navigation of Magnetic-Driven Microrobots Using OCT Imaging Feedback. IEEE Trans Biomed Eng. 2020 Aug;67(8):2349-2358. doi: 10.1109/TBME.2019.2960530. Epub 2019 Dec 18. PMID: 31869776.
Navab N, Bascle B, Loser M, Geiger B, Taylor R. Visual servoing for automatic and uncalibrated needle placement for percutaneous procedures. In: Proceedings IEEE Conference on Computer Vision and Pattern Recognition (CVPR); IEEE. 2000; 2:327-334.
Flückiger M, Neild A, Nelson BJ. Optimization of receiver arrangements for passive emitter localization methods. Ultrasonics. 2012 Mar 1;52(3):447-55.
Yan X, Zhou Q, Vincent M, Deng Y, Yu J, Xu J, Xu T, Tang T, Bian L, Wang YJ, Kostarelos K, Zhang L. Multifunctional biohybrid magnetite microrobots for imaging-guided therapy. Sci Robot. 2017 Nov 22;2(12):eaaq1155. doi: 10.1126/scirobotics.aaq1155. PMID: 33157904.
Ikemoto Y, Ito K, Sato K. Another Mechanism for Gait Generation: Mechanical Stabilization Can Spontaneously Realize Walking in a Two-Legged Robot.
Cho EC, Glaus C, Chen J, Welch MJ, Xia Y. Inorganic nanoparticle-based contrast agents for molecular imaging. Trends Mol Med. 2010 Dec;16(12):561-73. doi: 10.1016/j.molmed.2010.09.004. Epub 2010 Nov 10. PMID: 21074494; PMCID: PMC3052982.
Lee S, Chan Kwon I, Kim K. Multifunctional nanoparticles for cancer theragnosis. In: Nanoplatform-Based Molecular Imaging. 2011;541-63.
Kievit FM, Zhang M. Surface engineering of iron oxide nanoparticles for targeted cancer therapy. Acc Chem Res. 2011 Oct 18;44(10):853-62. doi: 10.1021/ar2000277. Epub 2011 Apr 29. PMID: 21528865; PMCID: PMC3192288.
Lee H, Shin TH, Cheon J, Weissleder R. Recent Developments in Magnetic Diagnostic Systems. Chem Rev. 2015 Oct 14;115(19):10690-724. doi: 10.1021/cr500698d. Epub 2015 Aug 10. PMID: 26258867; PMCID: PMC5791529.
Petrovic N, Petrovic MJ, Sreckovic S, Jovanovic S, Todorovic D, Vulovic TS. Nanotechnology in ophthalmology. Commercialization of Nanotechnologies–A Case Study Approach. 2018:275-97.
Johnston AP, Caruso F. Stabilization of DNA multilayer films through oligonucleotide crosslinking. Small. 2008 May;4(5):612-8. doi: 10.1002/smll.200700813. PMID: 18393261.
Guyer RA, Macara IG. Loss of the polarity protein PAR3 activates STAT3 signaling via an atypical protein kinase C (aPKC)/NF-κB/interleukin-6 (IL-6) axis in mouse mammary cells. J Biol Chem. 2015 Mar 27;290(13):8457-68. doi: 10.1074/jbc.M114.621011. Epub 2015 Feb 5. PMID: 25657002; PMCID: PMC4375497.
Dutta D, Sailapu SK. Biomedical applications of nanobots. In: Intelligent Nanomaterials for Drug Delivery Applications. Elsevier. 2020; 179-95.
Freitas RA. Nanotechnology, nanomedicine and nanosurgery. Int J Surg. 2005;3(4):243-6. doi: 10.1016/j.ijsu.2005.10.007. Epub 2005 Nov 28. PMID: 17462292.
Sivasankar M, Durairaj R. Brief review on nano robots in bio medical applications. Adv Robot Autom. 2012;1(101):2.
Rifat T, Hossain MS, Alam MM, Rouf AS. A review on applications of nanobots in combating complex diseases. Bangladesh Pharm J. 2019;22(1):99-108.
Li J, Esteban-Fernández de Ávila B, Gao W, Zhang L, Wang J. Micro/Nanorobots for Biomedicine: Delivery, Surgery, Sensing, and Detoxification. Sci Robot. 2017 Mar 15;2(4):eaam6431. doi: 10.1126/scirobotics.aam6431. Epub 2017 Mar 1. PMID: 31552379; PMCID: PMC6759331.
Wu Z, Li J, de Ávila BE, Li T, Gao W, He Q, Zhang L, Wang J. Water-powered cell-mimicking Janus micromotor. Adv Funct Mater. 2015;25(48):7497-501.
Wu Z, Wu Y, He W, Lin X, Sun J, He Q. Self-propelled polymer-based multilayer nanorockets for transportation and drug release. Angew Chem Int Ed Engl. 2013 Jul 1;52(27):7000-3. doi: 10.1002/anie.201301643. Epub 2013 May 23. PMID: 23703837.
Qiu F, Fujita S, Mhanna R, Zhang L, Simona BR, Nelson BJ. Magnetic helical microswimmers functionalized with lipoplexes for targeted gene delivery. Adv Funct Mater. 2015;25(11):1666-71.
Bozuyuk U, Yasa O, Yasa IC, Ceylan H, Kizilel S, Sitti M. Light-Triggered Drug Release from 3D-Printed Magnetic Chitosan Microswimmers. ACS Nano. 2018 Sep 25;12(9):9617-9625. doi: 10.1021/acsnano.8b05997. Epub 2018 Sep 11. PMID: 30203963.
Li J, Angsantikul P, Liu W, Esteban-Fernández de Ávila B, Thamphiwatana S, Xu M, Sandraz E, Wang X, Delezuk J, Gao W, Zhang L, Wang J. Micromotors Spontaneously Neutralize Gastric Acid for pH-Responsive Payload Release. Angew Chem Int Ed Engl. 2017 Feb 13;56(8):2156-2161. doi: 10.1002/anie.201611774. Epub 2017 Jan 20. PMID: 28105785; PMCID: PMC5511515.
de Ávila BE, Angsantikul P, Li J, Angel Lopez-Ramirez M, Ramírez-Herrera DE, Thamphiwatana S, Chen C, Delezuk J, Samakapiruk R, Ramez V, Obonyo M, Zhang L, Wang J. Micromotor-enabled active drug delivery for in vivo treatment of stomach infection. Nat Commun. 2017 Aug 16;8(1):272. doi: 10.1038/s41467-017-00309-w. Erratum in: Nat Commun. 2017 Oct 31;8(1):1299. doi: 10.1038/s41467-017-01616-y. PMID: 28814725; PMCID: PMC5559609.
Esteban-Fernández de Ávila B, Ramírez-Herrera DE, Campuzano S, Angsantikul P, Zhang L, Wang J. Nanomotor-Enabled pH-Responsive Intracellular Delivery of Caspase-3: Toward Rapid Cell Apoptosis. ACS Nano. 2017 Jun 27;11(6):5367-5374. doi: 10.1021/acsnano.7b01926. Epub 2017 May 5. PMID: 28467853; PMCID: PMC5894870.
Liu D, Yang F, Xiong F, Gu N. The Smart Drug Delivery System and Its Clinical Potential. Theranostics. 2016 Jun 7;6(9):1306-23. doi: 10.7150/thno.14858. PMID: 27375781; PMCID: PMC4924501.
Singh AV, Ansari MHD, Laux P, Luch A. Micro-nanorobots: important considerations when developing novel drug delivery platforms. Expert Opin Drug Deliv. 2019 Nov;16(11):1259-1275. doi: 10.1080/17425247.2019.1676228. Epub 2019 Oct 14. PMID: 31580731.
Chen C, Chen L, Wang P, Wu LF, Song T. Steering of magnetotactic bacterial microrobots by focusing magnetic field for targeted pathogen killing. J Magn Magn Mater. 2019;479:74-83.
Lin Z, Gao C, Wang D, He Q. Bubble-Propelled Janus Gallium/Zinc Micromotors for the Active Treatment of Bacterial Infections. Angew Chem Int Ed Engl. 2021 Apr 12;60(16):8750-8754. doi: 10.1002/anie.202016260. Epub 2021 Mar 9. PMID: 33481280.
Farahani A, Farahani A. An adaptive controller for motion control of nanorobots inside human blood vessels. Biosci Biotechnol Res Commun. 2016 Jul 1;9(3):546-52.
Manjunath A, Kishore V. The promising future in medicine: nanorobots. Biomed Sci Eng. 2014;2(2):42-7.
Biswas O, Sen A. Nanorobot the expected ever reliable future asset in diagnosis, treatment and therapy. In: Foundations and Frontiers in Computer, Communication and Electrical Engineering: Proceedings of the 3rd International Conference C2E2; 2016; 451.
Belachew GT. Disease fighting machines inside the body—A review. Biomed J Sci Tech Res. 2023;49(2):40454-62.
Kwan JJ, Myers R, Coviello CM, Graham SM, Shah AR, Stride E, Carlisle RC, Coussios CC. Ultrasound-Propelled Nanocups for Drug Delivery. Small. 2015 Oct 21;11(39):5305-14. doi: 10.1002/smll.201501322. Epub 2015 Aug 21. PMID: 26296985; PMCID: PMC4660885.
Pokki J, Ergeneman O, Chatzipirpiridis G, Lühmann T, Sort J, Pellicer E, Pot SA, Spiess BM, Pané S, Nelson BJ. Protective coatings for intraocular wirelessly controlled microrobots for implantation: Corrosion, cell culture, and in vivo animal tests. J Biomed Mater Res B Appl Biomater. 2017 May;105(4):836-845. doi: 10.1002/jbm.b.33618. Epub 2016 Jan 24. PMID: 26804771.
Tripathi PA, Singh AD. Natural resources from plants in the treatment of cancer: an update. Asian J Pharm Clin Res. 2017;10(7):13-22.
Preethi R, Padma PR. Anticancer activity of silver nanobioconjugates synthesized from Piper betle leaves extract and its active compound eugenol. Int J Pharm Pharm Sci. 2016;8(9):201-5.
Safdar MH, Hussain Z, Abourehab MAS, Hasan H, Afzal S, Thu HE. New developments and clinical transition of hyaluronic acid-based nanotherapeutics for treatment of cancer: reversing multidrug resistance, tumour-specific targetability and improved anticancer efficacy. Artif Cells Nanomed Biotechnol. 2018 Dec;46(8):1967-1980. doi: 10.1080/21691401.2017.1397001. Epub 2017 Oct 30. PMID: 29082766.
da Silva Luz GV, Barros KV, de Araújo FV, da Silva GB, da Silva PA, Condori RC, Mattos L. Nanorobotics in drug delivery systems for treatment of cancer: a review. J Mat Sci Eng A. 2016;6:167-80.
Yu J, Yang C, Li J, Ding Y, Zhang L, Yousaf MZ, Lin J, Pang R, Wei L, Xu L, Sheng F, Li C, Li G, Zhao L, Hou Y. Multifunctional Fe5 C2 nanoparticles: a targeted theranostic platform for magnetic resonance imaging and photoacoustic tomography-guided photothermal therapy. Adv Mater. 2014 Jun 25;26(24):4114-20. doi: 10.1002/adma.201305811. Epub 2014 Mar 26. PMID: 24677251.
Yu J , Chen F , Gao W , Ju Y , Chu X , Che S , Sheng F , Hou Y . Iron carbide nanoparticles: an innovative nanoplatform for biomedical applications. Nanoscale Horiz. 2017 Mar 1;2(2):81-88. doi: 10.1039/c6nh00173d. Epub 2016 Dec 1. PMID: 32260669.
Sun Z, Wang T, Wang J, Xu J, Shen T, Zhang T, Zhang B, Gao S, Zhao C, Yang M, Sheng F, Yu J, Hou Y. Self-Propelled Janus Nanocatalytic Robots Guided by Magnetic Resonance Imaging for Enhanced Tumor Penetration and Therapy. J Am Chem Soc. 2023 May 24;145(20):11019-11032. doi: 10.1021/jacs.2c12219. Epub 2023 May 16. PMID: 37190936.
Choi H, Lee GH, Kim KS, Hahn SK. Light-Guided Nanomotor Systems for Autonomous Photothermal Cancer Therapy. ACS Appl Mater Interfaces. 2018 Jan 24;10(3):2338-2346. doi: 10.1021/acsami.7b16595. Epub 2018 Jan 12. PMID: 29280612.
Orozco J, Pan G, Sattayasamitsathit S, Galarnyk M, Wang J. Micromotors to capture and destroy anthrax simulant spores. Analyst. 2015 Mar 7;140(5):1421-7. doi: 10.1039/c4an02169j. Epub 2015 Jan 27. PMID: 25622851.
Mellata M. Human and avian extraintestinal pathogenic Escherichia coli: infections, zoonotic risks, and antibiotic resistance trends. Foodborne Pathog Dis. 2013 Nov;10(11):916-32. doi: 10.1089/fpd.2013.1533. Epub 2013 Aug 20. PMID: 23962019; PMCID: PMC3865812.
Delezuk JA, Ramírez-Herrera DE, Esteban-Fernández de Ávila B, Wang J. Chitosan-based water-propelled micromotors with strong antibacterial activity. Nanoscale. 2017 Feb 9;9(6):2195-2200. doi: 10.1039/c6nr09799e. PMID: 28134392.
Li J, Angsantikul P, Liu W, Esteban-Fernández de Ávila B, Chang X, Sandraz E, Liang Y, Zhu S, Zhang Y, Chen C, Gao W, Zhang L, Wang J. Biomimetic Platelet-Camouflaged Nanorobots for Binding and Isolation of Biological Threats. Adv Mater. 2018 Jan;30(2). doi: 10.1002/adma.201704800. Epub 2017 Nov 28. PMID: 29193346.