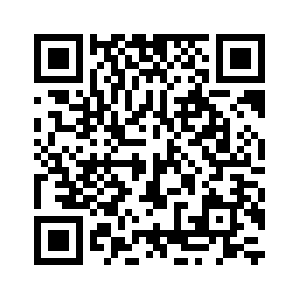
Mars Ascent Propellants and Life Support Resources - Take it or Make it?
Aerospace Engineering Energy Systems受け取った 16 Jul 2024 受け入れられた 26 Jul 2024 オンラインで公開された 29 Jul 2024
Focusing on Biology, Medicine and Engineering ISSN: 2995-8067 | Quick Google Scholar
Previous Full Text
A Study of Multi-Pose Effects On a Face Recognition System
受け取った 16 Jul 2024 受け入れられた 26 Jul 2024 オンラインで公開された 29 Jul 2024
Studies of Mars missions over the past thirty years lacked credible cost estimates, so the total mass of materiel delivered to Low-Earth Orbit (LEO) was typically used as a rough measure of relative mission cost because the complexity of the mission was thought to be roughly proportional to the initial mass in LEO (IMLEO). Historically, high launch costs led to large investments in space hardware development which led to high space mission costs. Reducing mass became the central theme of space mission engineering. We are now entering a new era where launch costs no longer have the impact that they would have two decades ago. Launch costs are coming down to the point where we must ask ourselves whether it now makes sense to bring ascent propellants and life support resources from Earth (with higher reliability as a bonus), as opposed to using in situ propellant production and cycling of life support resources.
Human missions to Mars have been under study for about 80 years. During that time, many mission studies were carried out by various groups and organizations. This history was described and discussed by several references [
, ].Studies of Mars missions over the past thirty years, lacking credible cost estimates, widely used the total mass of materiel delivered to LEO as a rough measure of relative mission cost because the complexity of the mission was thought to be roughly proportional to IMLEO. The term “initial mass in low Earth orbit” (IMLEO) became a common term in Mars mission planning [
]. In the very early days of space mission planning, launch costs to LEO might have been as great as $50,000 per kg, and later more likely $20,000/kg. If, as seems likely, IMLEO for a human mission to Mars might be say, 1,200 tons, and if the cost of delivery from Earth to LEO was say, $20,000 per kg, then the mission cost for launch to LEO would be $24 billion – which is significant. In that era, investment in technology to reduce IMLEO was well worth it.Jones (2024) suggested that launch costs will drop below $1,000/kg with the advent of Starship [
]. He pointed out that prior to the last decade or two, launch cost was the major impediment to exploring and exploiting space. High launch costs led to high investment in space hardware development which led to high space mission costs. When the gear sent into space cost $50,000 per kg to launch to LEO, each kg of payload was precious, and reducing mass became the central theme of space mission engineering. Investing to reduce mass by a kilogram was worth it. As Jones put it: “Spacecraft designers and space mission planners are mass misers”. Engineers shun “brute force” approaches and seek creative methods to reduce mass. The ingenuity with which engineers reduce mass is admirable, but we are now entering a new era where this no longer has the impact that it would have two decades ago. Jones provided the allegory of a poor person who suddenly becomes rich but continues to count his pennies and lives frugally.As we entered the 21st century, the space establishment was driven by the fundamental belief that reducing mass was a primary objective of space technology. This is evidenced by the fact that the important NASA study of human missions to Mars known as “Design Reference Architecture-5” (DRA-5) placed emphasis on reducing mass, specifically reducing IMLEO. In this document, slide 10 is completely devoted to estimates of IMLEO, slide 18 compares mission options for their relative values of IMLEO, and slides 21-25 and slide 27 are concerned with reducing total mission mass. In their recommendations for “forward work” (slide 47), they said “Address options for reducing total mission mass and thus a number of launches”. Reducing mass is always a good thing in principle, but when the means of reducing mass introduces higher cost than launch cost, as well as a dependence on remote autonomous systems that add risk and questions of reliability, the trade might not favor reducing mass [
].If, as seems likely, IMLEO for a human mission to Mars might be say, 1,200 tons, and if the cost of delivery from Earth to LEO was say, $20,000 per kg, then the mission cost for launch to LEO would be $24 billion – which is significant. In that era, investment in technology to reduce IMLEO was worth it. With launch costs a factor of twenty lower, the equation has changed.
Two important subsystems for Mars missions are ascent propellants for return from Mars, and life support (providing water and breathing air to the crew). If these were delivered from Earth, both would require large masses of materiel on Mars. Therefore, in the era of high launch costs, engineers devised in situ propellant production (ISPP) to produce propellants for ascent from indigenous resources on Mars, and recycling systems for air and water. Both technologies significantly reduce IMLEO. However, both technologies require long-term autonomous operation of complex systems which add significant cost to overcome inherent risk, and with the advent of low launch cost, it might be less expensive and more robust to bring these resources from Earth [
].The critical factors in large-scale space missions such as humans to Mars are cost and risk. Many components and subsystems contribute to overall risk, so each one must achieve a very high reliability. Because risk is difficult to estimate, it has not usually been adequately considered in evolving new technologies. The cost has been mainly based on the reduction of IMLEO, which made some sense in the era of high launch costs. The two technologies considered in this paper: ISPP and cycling in life support, were both put forward mainly to reduce IMLEO, but they introduced significant risks. Furthermore, the cost to prospect or validate on Mars is typically high, and when compared to the cost and risk of bringing resources from Earth, the basis for these technologies weakens in the era of low launch costs.
Launch logistics must also be considered. Even though launch costs might be low, if one follows the option of bringing resources from Earth, the need to launch large masses might cause a logjam in launches. The advent of a super-heavy launch vehicle would help in this regard.
The approach taken in this paper is as follows.
First, the mission scenarios are briefly reviewed for human exploration of Mars.
Next, the expectations for reductions in launch costs in the next decade are reviewed. That is necessarily somewhat subjective because the state of the technology is in flux with costs dropping sharply, but it is not necessarily clear how far they will drop or how those reductions relate to the size of the payload. Most of the information on future launch costs has been published as news reports, rather than serious technical papers.
Next, the technologies for ISPP on Mars are reviewed with emphasis on the cost to develop and validate on Mars and rough appraisals of risk involved in the operation of autonomous systems for many months. While the ISPP community did a good job of describing the mass-saving benefits of ISPP in the era of high launch costs, there is a serious lack of consideration of costs to validate on Mars, and prospecting (where needed), and risk seems not to have been considered at all. ISPP is divided into two parts. ISPP that processes only the atmosphere has lower cost and less risk than processes that require autonomous digging up regolith, transporting regolith, and inserting and removing regolith in reactors, many hundreds of times. Rough estimates of costs and risks for both forms of ISPP are compared with the launch cost for bringing ascent propellants from Earth.
Then, the requirements for life support on long missions are reviewed. Surprisingly, the requirements for daily water per crewmember have not been clearly discussed and evaluated by the life support community. The technology for cycling derives from the system presently on the International Space Station (ISS) which requires frequent “orbital replacement units” for subsystems that use up resources or fail. Using known requirements for air, and reasonable estimates for water, the requirements and costs to bring these from Earth and compare risk and cost to strategies for reducing risk of cycling systems are determined. The optimum choice might be to bring basic survival resources from Earth and use cycling to upgrade the water supply.
Finally, the results are summarized and further work needed to clarify the neglected topics of cost and risk for ISPP in large-scale Mars missions are pointed out.
Scenarios for human missions to Mars were discussed at length by Rapp [
]. A so-called short-stay mission is viewed as the lowest cost option, where the crew stays on Mars for less than a month getting acclimated and preparing for return. This approach was rejected by NASA system engineers [ ] and was shown to lack significant exploration value by Rapp [ ]. A long-stay mission provides a great opportunity for exploration of Mars. It includes delivery of the crew to Mars, delivery of an Earth Return Vehicle (ERV) to Mars orbit, and a Mars Ascent Vehicle (MAV) to rendezvous with the ERV when it is time for the crew to return to Earth. In such a mission:About 40 tons of propellants are required on Mars for the MAV holding a crew of six. ISPP was envisaged as a means of greatly reducing IMLEO by producing propellants on Mars rather than bringing them from Earth.
The crew requires life support, particularly water and breathing air on the journey to Mars, while on Mars, and on the return trip to Mars. The required amount of water is massive, and NASA has been working on cycling systems for many years to avoid bringing it all from Earth. This would require three cycling systems, two operating in zero-g and one operating in 0.3 g.
A great many news releases and commentaries have appeared on the Internet in the past few years pointing out the great reductions in launch cost to LEO now taking place via progress made by Space X, with further reductions predicted for the future. Such postings on the Internet, while not necessarily reliable, predict future launch costs down to $100/kg to LEO with Starship [
].Weinzieryl, et al. (2024) provided an excellent history and a review of 2024 launch costs. They said Space X currently offers launches on Falcon 9 at $2,000 per kg to LEO and Falcon heavy at about $1,300 per kg to LEO [
].Apparently, Jones (2018) was the first in the NASA community to point out the implications of this for future NASA space missions. [
] He pointed out that the Falcon 9 reduced the cost to LEO to $2,700 per kg and discussed projections for significant further reductions in the future. In addition, the Internet suggests that a super-heavy launch vehicle that can lift 200 to 300 tons might be under development at Space X but this remains speculative.The gear ratio for the transfer of a payload from one destination to another destination is the total initial mass divided by the payload delivered. The initial mass includes the payload, the propulsion system, and the propellant. The gear ratio for transfer from LEO to Mars locations was estimated by Rapp (2023) [
]. Using full aero-assist and H2–O2 for departure from LEO, he obtained the data in Table 1. For example, the mass of a landed payload should be multiplied by 10.5 to estimate IMLEO to land that payload on Mars.The use of indigenous resources on Mars for a variety of applications is an appealing approach to reduce IMLEO, and for visionaries, to provide a basis for long-term settlements. This concept originated in a landmark paper published in 1978 and has been included in mission studies since the 1990s [
]. Since the 1990s, Sanders has been a leading NASA advocate for “in situ resource utilization” (ISRU) of which production of propellants for ascent from Mars (ISPP) is a near-term application with a large potential impact on IMLEO. Sanders has many advocacy publications with many approaches to utilizing remote resources, and here only one publication is cited as an example [ ].ISPP was reviewed and analyzed at length by Rapp [
, ]. He showed that roughly 40 tons of propellants would be needed for a crew of six to ascend from Mars to rendezvous with the Earth Return Vehicle (ERV) waiting in Mars orbit. A circular orbit is used if ISPP is employed, while an elliptical orbit is used if propellants are brought from Earth. The propellant requirement depends exponentially on the change in velocity (“delta-v”) that must be imparted to the ascent vehicle to rendezvous with the ERV.CH4 and O2 propellants are the most likely propellants for ascent. If only the CO2 atmosphere is processed, O2 is produced (representing about 78% of total propellant mass). The advantage is that the atmosphere-only ISPP system can be placed almost anywhere on Mars, and there are no moving parts except the compressor to draw in Mars’s atmosphere. No prospecting is required.
To produce both CH4 and O2, large amounts of regolith must be processed to obtain water, either from ice or mineral hydrates, which involves remote autonomous processes repeated many hundreds of times that introduce serious risk considerations. Prospecting to find the best location will require multiple exploration missions to Mars driving up the cost.
All ISPP processes are power-hungry, and demonstrating and validating them on Mars requires large multi-kW power systems that drive up the cost.
In the era of high launch costs, the costs to develop, prospect, validate, and implement ISPP were assumed to be less than launch costs to bring from Earth, but details were not investigated. In review, these costs now loom large, particularly for processes that require regolith.
The change in velocity (delta-v) for liftoff is 4.3 km/s to a 500 km circular orbit and 5.6 km/s to a 1-sol elliptical orbit. Because of the exponential dependence of ascent propellant requirements on delta-v, this makes a significant difference. Using CH4 and O2 propellants with specific impulse (Isp) ~ 360 s, the ascent propellant requirements are estimated for a crew of six as follows:
If ascent propellants are brought from Earth, the circular orbit would be utilized. The mass required in LEO is estimated as 10.5 x 34.1 = 358 tons.
If ascent propellants are produced by ISPP, the elliptical orbit is chosen for the ERV, and there are two possibilities:
The above discussion is oversimplified. The mass of tankage, cryocooling, and any other ancillary equipment in the transfer to Mars was not included. No allowance was made for the large power systems for ISPP and the smaller power systems for cryogenic storage. If most of this power for ISPP can be rerouted to life support when the crew arrives, the power system is not attributable to ISPP since it would be needed for life support even if ISPP was not utilized. However, if the ISPP power level exceeds that for life support, this would require further review. It is more likely that the higher power level associated with producing both CH4 and O2 would be greater than the need for life support when the crew arrives, so this might add significantly to the mass attributed to ISPP in that case.
In summary, the masses (tons) are shown in Table 2.
Now, the question arises as to what is the cost to develop, prospect, validate (on Mars), and implement each form of ISPP. There is no need to estimate this exactly (indeed that would be very difficult at this stage) but to roughly characterize the cost of ISPP relative to the $358 million launch cost (at $1,000/kg) for bringing propellants from Earth.
In the evolution of an ISPP system from proof-of-concept, to pilot stage, to scaled system, to full-scale system, account must be taken of the fact that ISPP is power hungry and even a small pilot version requires considerably more continuous power than can be supplied by an RTG. A very important question, not yet addressed by NASA despite the many advocacy papers for ISPP by NASA, is how extensive a demonstration at what scale must be validated in situ on Mars, and how would power be provided? It seems clear that any ISPP demonstration on Mars will require a significant continuous power system which immediately indicates costs in the billions, well beyond the $358 million figure for the “take it” option. Even the simple MOXIE project that rode “piggyback” on a Science Rover and ran intermittently at less than 1% of full-scale cost over $50 million. Validation of the option of atmosphere-only ISPP will require at least several billion dollars. The option includes processing regolith to obtain water, multiple missions to Mars for prospecting and validation at several scales, and the runout cost might be more than $10 billion, perhaps considerably more than that. While a detailed cost estimate of the prospecting and validation processes based on a sequence of landings on Mars and observations from orbit hesitates the author to be attempted, it seems evident that the “take it” option for ascent propellants on Mars is far less expensive and far more robust with far less risk than any form of ISPP, particularly the ISPP approach that requires autonomous processing of regolith.
This suggests the following assumed costs and risks in Table 3. The costs to develop, prospect, validate, and implement ISPP are difficult to estimate at this time, but they will require several landings on Mars with significant power systems. A guess is hazarded that the cost for atmosphere-only ISPP would be $5 billion and the cost of ISPP utilizing regolith would be $10 billion. The exact amounts do not matter if these figures are in the right “ballpark”.
At the bottom line, the launch costs are small compared to the costs for development, prospecting, validation, and implementation of ISPP. Bringing propellants from Earth is the lowest cost and least risky. However, the mass transported to LEO for ascent is estimated to be 358 tons and this is likely to present logistic launch problems if a super heavy launch vehicle is not available.
Life support requirements: A human crew requires an Environmental Control and Life Support System (ECLSS) to maintain the health and robustness of the crew on long missions. The most fundamental important data for life support on long missions is the requirement (kg per crew member per day) for breathing air and water. The mass requirement for water far exceeds that for air.
There is widespread agreement that the requirement for breathing air is around 1 kg/CM/day. For a 200-day trip to or from Mars, 200 kg would be needed each way. For 500 days on Mars, 500 kg would be needed. The total air requirement is 900 kg, which is rounded off to about a ton.
The water requirement for long-duration missions has not been clearly defined, explained, and reviewed by NASA. While many papers extol the virtues of cycling, the basic requirement for various duration missions remains vague and unclear. Before anyone writes about life support, they should first discuss how much water is needed, and what privations would be endured at various levels of water supply in long missions, and as far as the author can tell, this has not been done. There are a few papers here and there that specify some allocation of water (kg/CM/day) but none of these explain how these were derived or what level of comfort and/or privation for the crew would be associated with any level. They are typically single entries in a table. Furthermore, the huge allocation for laundry water in some studies seems excessive. Bringing replacement clothes, and taking steps than other water laundry might significantly reduce this water requirement. For example, tumble-drying in a warm chamber open to Mars ambient might do a good job at removing volatiles and microbes.
One of the better review articles on life support is Hanford (2004) [
]. Hanford provides tabular data, but like all life-support publications, it has been found that Hanford does not provide any backup or support for his figures. His data is given to 3 significant figures, yet they only seem credible to at most, 1.5 figures. It is not clear why he allocates 0 to dishwashing, and the laundry requirement seems incredibly high. Reference [ ] suggests 5.4 kg/CM/day for dishwashing. Hanford assumed that neither dishwashing nor laundry would be used for an “early planetary base” but would be used for a “mature planetary base”. Since even an early Mars base would entail ~400 days of travel and ~500 days on Mars, even an early Mars base would seem to require dishwashing and laundry. The tradeoff between throw-away clothes and laundry was not discussed. If the allocation of 2.0 kg/CM/day is for dishwashing, the daily total is 9.7 kg/CM/day without laundry and dishwashing, and 24.2 kg/CM/day with laundry and dishwashing (Table 4).Allen, et al. (2004) distinguished between potable water used for drinking, food preparation (typically food rehydration in prior missions) and oral hygiene [
]. Hygiene water was primarily “for external use, such as body cleansing, and as with the potable water, cannot be contaminated with high levels of contaminants.” Table 5 summarizes the amount of water allotted per crew member per day for different operational states. It is difficult to interpret this table. The terms off-nominal and degraded are not explained. The “hygiene nominal” figure seems high. It is amazing that NASA could put out a report this this important basic data and provide no context or backup for the cryptic table entries. Twenty years later, we are not much better off.More recently, Jones (2020) discussed life support requirements based on experience with Space Station Freedom (SSF) and the International Space Station (ISS) [
]. The two major needs are for breathing air and water. The air requirement is about 1 kg/CM/day in agreement with all other findings. The water requirement requires discussion. Jones began with the results of Reference 22 with two modifications:Since Jones was interested in delivering mass from LEO, he multiplied the amount of water used by a packaging factor of about 1.2 to obtain the mass that would have to be delivered from Earth.
Jones attributed the data in Table 4 to design requirements for SSF. He pointed out that the habitable conditions for ISS were more stringent and the water allocations for showers, clothes washing, and dishwashing were eliminated on ISS, reducing the water requirement to ~7 kg/CM/day. He then distinguished between the ISS water requirement of 7 kg/CM/day and “a more comfortable Earth-like environment” with a water requirement of 27.6 kg/CM/day.
Obviously, one can imagine a range of possible options for water consumption on a Mars mission intermediate between the austere survival level of 7 kg/CM/day and the more luxurious Earth-like 27.6 kg/CM/day, but it seems likely that a 900-day mission would require “a more comfortable Earth-like environment”. Nevertheless, Jones’ figure of 27.6 kg/CM/day seems bloated by the high laundry and dishwashing allocations recommended by his predecessors. Here, the figures shown in Table 6 are adopted, with a figure of 16.7 kg/CM/day attributed to “a more comfortable Earth-like environment”.
Options for providing water and air to the crew: In the analysis that follows, the two extremes for water (7 and 16.7 kg/CM/day) are utilized, and the consequences for “take it or make it” are discussed for these two end-points with the understanding that one might interpolate between them.
As shown previously, the air requirement is fairly certain at about 1 kg/CM/day. The water requirement remains uncertain and is assumed that a minimal survival level is 7.0 kg/CM/day, while a comfortable Earth-like environment would be provided by 16.7 kg/CM/day. Packaging is not included here because it is needed in both “take it” and “make it” modes. A Mars mission with a crew of six, 200 days of travel each way to and from Mars, and 500 days on the surface is considered. In any case, the requirement for air is the same:
With a comfortable Earth-like water environment, the water requirements are:
With a minimal but easily survivable water level, the water requirements are:
It can then conceive the following modes of life support:
1. Bring air and a comfortable Earth-like environment water from Earth.
The total required water and air mass on the ERV = 42.5 tons
The total required water and air mass on Mars = 53.1 tons
IMLEO is roughly estimated to be 830 tons
2. Fully utilize recycling for a comfortable Earth-like environment.
The masses involved in this case are the masses of the recycling systems plus a backup cache to allow for losses. A wild guess is that the Mars recycling system and the transit recycling system (including backup cache) would each have a mass of 6 tons including backup. IMLEO is roughly estimated to be 100 tons. This is a great reduction in IMLEO compared to bringing resources from Earth, but it makes the mission reliability dependent on the continuous performance of the cycling systems over 900 days.
3. Bring air and survivable water from Earth.
In this option, water and air are brought from Earth but the crew, both in transit and on Mars, is required to live with a survivable level of water (7.0 kg/CM/day).
The total required water and air mass on the ERV = 19.2 tons
The total required water and air mass on Mars = 24.0 tons
IMLEO is roughly estimated to be 375 tons
4. Bring all breathing air and easily survivable water from Earth, and use cycling to enhance water to a comfortable Earth-like environment.
In this case, all the breathing air from Earth is brought over plus enough water from Earth to meet the easily survivable level (7.0 kg/CM/day). Cycling is used to increase the average water use rate to 16.7 kg/CM/day. The mass of the cycling system is reduced to 5 tons.
The total required recycle system, water, and air mass on the ERV = 24.2 tons
The total required recycle system, water, and air mass on Mars = 29.0 tons
IMLEO is roughly estimated to be 460 tons
This approach provides easily survivable water with high reliability and allows an upgrade to water in a comfortable Earth-like environment using a (less reliable) cycling system.
As a guess, it is assumed that the cost to develop a full-scale water and air recycling system including a full-scale demonstration on Mars prior to human landing would be $8 billion, and a 2/3 size system would cost $6 billion. This suggests the costs (billions) and risks in Table 7. Reference 4 suggested that the cost might be closer to $2 billion. That might well be true for development, but full-scale demonstration on Mars would probably cost more than $2 billion. The arguments given below do not depend that much on the actual cost as long as it is several billion.
The most expensive option (#2) is to fully recycle to an Earth-like environment at a cost of about $8.1 billion. The risk is much higher than other options because life is dependent on the continuous operation of cycling systems for 900 days. Bringing all resources from Earth (#1) to establish a comfortable Earth-like environment is very affordable but IMLEO is so high that the logistics appear to be formidable. Option #4 is the most attractive.
It is a mathematical truism that two curves, one rising and the other dropping must cross at some point. As launch costs come down and NASA plans for ISPP become more elaborate, a point must be reached where bringing resources from LEO makes more sense (in terms of cost and risk) than investing in remote autonomous technology that is inherently risky.
After examination of the in situ propellant production and life support from the point of view of whether it is beneficial to bring resources from Earth or generate them in situ on Mars. The three important criteria are:
Assessing cost, risk, and launch logistics presents a challenge for various options for future large-scale human missions. Despite the uncertainty, one major assumption stands out. Here, it is assumed that bringing resources from Earth reduces risk, and any autonomous system operating for many months far from Earth is inherently riskier – in our opinion, a great deal riskier. It is not clear how much risk can be tolerated in such missions and planners for ISPP and life support have not developed credible estimates of the costs and risks inherent in their technologies. For a human mission that depends on many subsystems, each one must reach a level of low risk not attained in robotic missions.
The advocates for ISPP seem to be locked into the old paradigm of reduction of IMLEO as the principal objective, regardless of cost and risk [
]. It is not clear what tests, prospecting missions, and verification procedures on Mars would be necessary to validate ISPP before sending a crew to Mars – whose lives depend on ISPP. This seems to be remote territory in the NASA universe. All ISPP systems are power-hungry. Even a scaled-down system would require more than 10 kW continuous for a long-duration test [ , ]. An autonomous atmosphere-only system is inherently less risky than one that utilizes regolith because it can be dropped down almost anywhere on Mars, connected to a power system, turned on, and allowed to run. An autonomous ISPP system that requires excavation, digging, and transporting regolith, inserting and removing regolith from a reactor, dumping spent regolith, and doing this many hundreds of times faultlessly in a remote, harsh environment, is inherently subject to more risk [ , ]. Prospecting to find suitable regolith deposits will require multiple orbital and ground truth searches costing multiple billions of dollars. It might be argued that the crew would not launch until the ISPP system completed its job and filled the tanks of the ascent vehicle prior to the crew launch date, thus preventing disaster for the crew [ , ] but that provides a false sense of security. If the entire crew and cargo launch system, representing an investment of perhaps two hundred billion dollars or more, is lined up and ready to go, and the ISPP system fails prior to crew launch, the crew is saved by not launching but the entire assemblage of Mars mission preparations is thrown out of schedule and the financial losses would be huge.For ascent propellants, the issue is clear. The use of ISPP in any form is less attractive than bringing the propellants from Earth. The cost is the lowest and the risk is lowest for bringing ascent propellants from Earth. The only negative factor is that IMLEO is much higher at 358 tons. In the era of super heavy launch vehicles that could probably be accommodated but it does introduce logistic challenges.
The issue of life support is fraught with considerable uncertainty. The state of the technology of air and water cycling is not very clear. Even the requirements for water are nebulous. There are merits and faults of the system used on the ISS but the requirement for frequent replacement of components and subsystems indicates a significant level of unreliability [
]. Reliable cycling systems do not seem to exist. The technologies involved in the ISS system might be a starting point for a more reliable and robust system design. To that end, Jones and co-workers published a series of papers analyzing the use of redundancy and spares to shore up the reliability of the fragile system. (For example, [ ]). Owens, et al. (2020) estimated various masses and mentioned “risk” and “reliability” several times but they did not estimate risk or reliability. Their study included the use of spares, but the author was unable to discern at what level or how reliability was affected by spares [ ]. Broylan, et al. (2021) provided a summary of NASA’s plans for developing advanced closed life support systems. They stated the goal was to achieve 99% reliability using spares. And while “reliability” was mentioned in about 20 places in the document, these statements only emphasized NASA’s awareness of the need. No data was presented, nor was a plan to measure reliability even defined [ ]. It is not clear what level of reliability will be needed or how it will be measured. Presumably, it would involve some combination of lab testing and validation on Mars. But how many parallel systems would be tested in the lab with what human participation, and how can one test on Mars with humans? There seems to be a built-in discrepancy. One cannot validate without humans participating on Mars and one cannot send humans to Mars without validation. Jones modeled the number of redundant units needed to achieve any required redundant reliability and confidence level. This depends on the measured system failure rate, the mission length, the required redundancy reliability, and the required confidence in that reliability [ ]. Since data for insertion in this formalism is sketchy, it is difficult to make practical use of it. Owens, et al. (2022) carried out a complex mathematical analysis of a plan to test and develop in parallel – to gradually improve the technology to reduce the number of spares [ ]. However, reducing the number of spares might not be that valuable compared to bringing resources from Earth because the risk remains. The test/development plan was not actually described or cost-estimated and this paper seems to be more of a demonstration of mathematical prowess than a viable plan to make life support reliable.Another important aspect of life support is the water requirement. As discussed previously, Jones suggested that there is a minimum survivable water level (7.0 kg/CM/day) and a level of Earth-like comfort (he suggested 27.6 kg/CM/day but used 16.7 kg/CM/day). The impact of the level of the water supply level on the crew’s health, psychological robustness, and overall mission success has not been investigated or understood.
The author roughly estimated cost, risk, and logistic feasibility for four options involving cycling or bringing resources from Earth, or a combination of the two. In order to minimize risk and provide Earth-like comfort for a long-duration human mission to Mars, the hybrid is recommended, where survival resources are brought from Earth and cycling is used to increase life support to a level of Earth-like comfort.
Several important assumptions were made in this study. They can be justified to some extent as being credible, but they do not have a strong underpinning in fact. In matters of cost and reliability/risk of evolving technologies involving remote, autonomous operations many hundreds of times, some educated guesswork is necessarily involved.
Assumption 1: It was assumed that the mass of a recycle system including a backup cache is 6 tons. The mass of a recycling system is anyone’s guess, but the backup cache is likely several percent of the total water requirement which is 90 tons. In any event, it does not matter whether the mass is 3, 6, or 9 tons because IMLEO is comparatively low with recycling, independent of the assumed mass.
Assumption 2: It was assumed that the launch cost would drop to $1,000 per kg to LEO. That has been widely predicted (Reference 5 for example). But even if the author used $2,000, none of the conclusions in this paper would change.
Assumption 3: It was assumed that the cost to develop, prospect (where applicable), demonstrate, and validate on Mars an ISPP system would be $5 billion for an atmosphere-only ISPP and $10 billion for an ISPP system that required autonomous processing of regolith (either for ice or minerals with hydration). Nobody knows the cost of such enterprises. However, it is known that ISPP systems are power-hungry, and demonstration/validation on Mars would require well over 10 kW continuous even for a scaled-down system. Validation on Mars would require such continuous elevated power levels for long durations. The power system alone might account for the cost estimates. The starting cost for almost any mission to Mars is a billion dollars. Prospecting for ice or minerals, ground truth, demonstration/validation of autonomous regolith gathering, transportation, insertion and removal from the reactor, and processing will require several landings on Mars and a significant power source. Prospecting will likely require multiple missions to Mars orbit and the Mars surface.
Assumption 4: It was assumed that the cost to develop, demonstrate, and validate on Mars a recycling system would be $8 billion. This, the author admits is rather a wild guess. There seems to be a total lack of any thinking, planning, and estimating in the NASA community of what it would take to validate a cycling system, first in the lab and then on Mars. How many units in parallel for how long, and what human inputs would be needed? Validation on Mars remains a total blank. If we do not first test it with humans on Mars, how can we send humans to Mars to use it?
Assumption 5: It was assumed that bringing any resource from Earth is less risky than using autonomous processing on Mars (ISPP or cycling). This seems evident to this writer, but it is a complex subject requiring further analysis.
Since the landmark paper by Ash, et al. (1978), ISPP has been a topic of interest in the NASA community. Jerry Sanders and Diane Linne have been tireless internal advocates for ISRU within NASA for a long time. (e.g. [
]). The unstated (but implied) justification for this was a reduction in IMLEO by producing ascent propellants in an era of high launch costs. Thus, there seems to be very little published analysis on costs for development, validation, prospecting, and implementation, and essentially nothing on overall risks in dependence on remote autonomous systems that must function repeatedly many hundreds (or thousands) of times in a harsh environment. Validating these power-hungry systems on Mars would be expensive. For systems requiring prospecting, costs are even higher. With the great reduction in launch costs, the reduction of IMLEO no longer constitutes a solid basis for ISPP and the option to bring ascent propellants from Earth becomes competitive. In this paper, the author has compared options for atmosphere-only ISPP, ISPP with processing regolith, and bringing ascent propellants from Earth based on cost and perceived risk. The author concluded that bringing ascent propellants from Earth is the least risky and least expensive but it might create launch logistics unless a super-heavy launch vehicle is available. Processing regolith and atmosphere has the highest cost and highest risk.NASA developed the ECLSS system now in use on the ISS. This system is composed of a number of subsystems and components. Some require periodic replenishment of resources; others break down occasionally and must be replaced by so-called “orbital replacement units” [
, ]. NASA does not appear to have a plan to adapt this system to the requirements of a human mission to Mars requiring 900 days of life support. The statistics for providing spare units were discussed by Jones in several papers (e.g. [ ]). One idea that was suggested was a test and upgrade program over many years to attempt to achieve a more robust system, but that seemed to bog down in mathematics and it is not clear how or whether it would work [ ]. Testing such systems will be a challenge because they require human participation and that would be difficult in situ on Mars. The best solution appears to be to provide low-risk survival levels of water from Earth and use riskier cycling (with replacement units) to upgrade the water supply to more satisfactory levels.While this paper was restricted to Mars, it is somewhat extendable to the Moon. NASA has ambitious plans for lunar ISPP [
, , ]. For example, Reference 18 considered systems processing up to 680 tons of regolith per day. Very complex autonomous ISPP processes in harsh locations are now being proposed for the Moon. They appear to be far riskier than the risk associated with simply bringing propellants from Earth [ ].Another concept that has emerged in the 21st century is the notion of a “Gateway” in cis-lunar space [
]. The Gateway was designed to serve logistic functions in transfers to and from the Moon. However, Zubrin (2019) argued that it is unnecessary because, with the advent of new low-cost launchers, one can fly directly from LEO to the lunar surface, saving many years of development, and many billions of dollars [27]. While this is not exactly a question of “take it or make it”, it is the advent of low-cost, heavy-lift launchers that supports Zubrin’s arguments for direct transfer.NASA has clearly lagged in considering the recent progress in reducing launch costs on the trade between bringing resources to Mars and producing them in situ and remains locked in the paradigm of reducing IMLEO regardless of risk and cost. This paper does not provide the end-all of the discussion of this very important topic of “take it or make it”. Further analysis and discussion will undoubtedly evolve. But this paper should be taken as a “wake-up call” to NASA to begin thinking about the bigger picture of providing resources for human missions to the Moon and Mars.
Rapp D. Human Missions to Mars. 3rd ed. Heidelberg, Germany: Springer-Praxis Books; Springer.
Genta G. Next Stop Mars. Heidelberg, Germany: Springer-Praxis Books; Springer; 2016.
Jones HW. The recent large reduction in space launch cost. In: 48th International Conference on Environmental Systems; 2018 Jul 8-12; Albuquerque, NM. Paper ICES-2018-81.
Drake BG. Mars design reference architecture 5.0 study - executive summary. 2008. Available from: https://www.nasa.gov/wp-content/uploads/2015/09/373669main_2008-12-04_mars_dra5_executive_summary-presentation.pdf?emrc=db5841
Jones HW. Take material to space or make it there? In: 2023 ASCEND Conference. Las Vegas, NV. 2023.
Space Ambition. The Starship: can one rocket change the entire space industry? 2024. Available from: https://spaceambition.substack.com/p/the-starship-can-one-rocket-change
Weinzieryl MC, Lucas K, Sarang M. Space X, economies of scale, and a revolution in space access. Harvard Business School Report 9-720-027; 2021.
Ash RL, Dowler WL, Varsi G. Feasibility of rocket propellant production on Mars. Acta Astronautica. 1978;5:705-724.
Sanders G, Kleinhenz J. In situ resource utilization (ISRU) "Envisioned future priorities". In: Space Resources Roundtable (SRR). Golden, CO. 2022.
Rapp D. Use of extraterrestrial resources for human space missions to Moon or Mars. 2nd ed. Heidelberg, Germany. Springer-Praxis Books; Springer. 2018.
Hanford AJ. Advanced life support research and technology. NASA Report NASA/CR-2004-208944C. 2004.
Hanford AJ. Advance life support baseline values and assumptions. NASA Report NASA/CR-2004-208941. 2006.
Allen CS, Burnett R, Charles J, et al. Guidelines and capabilities for designing human missions. NASA Report NASA/TM-2003-210785. 2003.
Jones H. Minimum risk space habitat and life support. In: 2020 International Conference on Environmental Systems. 2020; Paper ICES-2020-222.
Rapp D, Inglezakis V. Mars in situ resource utilization (ISRU) – a historical review and appraisal. Appl Sci. 2024;14:653.
Rapp D. Near term NASA Mars and lunar in situ propellant production (ISPP): complexity vs. simplicity. Space Sci Technol. 2024.
Bagdigian RM, et al. International space station environmental control and life support system mass and crewtime utilization in comparison to a long duration human space exploration mission. In: 45th International Conference on Environmental Systems. Seattle, WA. 2015; Paper ICES-2015-094.
Jones HW. Redundancy: how many unreliable spares are needed for high reliability and confidence on a time-limited mission? Personal communication; 2021.
Owens AC, et al. Integrated trajectory, habitat, and logistics analysis and trade study for human Mars missions. In: ASCEND 2020. Virtual. 2020.
Broyan JL, et al. NASA environmental control and life support technology development for exploration: 2020 to 2021 overview. In: 50th International Conference on Environmental Systems. 2021; Paper ICES-2021-384.
Owens AC, Cirillo WM, Piontek N, et al. Analysis and optimization of test plans for advanced exploration systems reliability and supportability. In: 50th International Conference on Environmental Systems; 2021; Paper ICES-2020-199.
Sanders G, Kleinhenz J, Linne D. NASA plans for in situ resource utilization (ISRU) development, demonstration, and implementation. Presentation to COSPAR. 2022. Available from: https://ntrs.nasa.gov/api/citations/20220008799/downloads/NASA%20ISRU%20Plans_Sanders_COSPAR-Final.pdf
Jones HW. Life support with failures and variable supply. In: 40th International Conference on Environmental Systems. Barcelona, Spain. 2010.
Using space-based resources for deep space exploration. 2024. Available from: https://www.nasa.gov/overview-in-situ-resource-utilization/
Elliott J, Sherwood B, Austin A, et al. ISRU in support of an architecture for a self-sustained lunar base. In: 70th International Astronautical Congress (IAC); Washington, DC. 2019. Paper IAC-19-D3,2A,2,x51412.
Zubrin R. Op-ed | Lunar Gateway or Moon Direct? Space News. 2019. Available from: https://spacenews.com/op-ed-lunar-gateway-or-moon-direct/
Rapp D. Mars Ascent Propellants and Life Support Resources - Take it or Make it? IgMin Res. July 29, 2024; 2(7): 677-686. IgMin ID: igmin232; DOI: 10.61927/igmin232; Available at: igmin.link/p232
次のリンクを共有した人は、このコンテンツを読むことができます:
Address Correspondence:
Dr. Donald Rapp, Mechanical and Chemical Engineering, Caltech - JPL (retired), 1445 Indiana Avenue, South Pasadena, CA 91030, USA, Email: [email protected]
How to cite this article:
Rapp D. Mars Ascent Propellants and Life Support Resources - Take it or Make it? IgMin Res. July 29, 2024; 2(7): 677-686. IgMin ID: igmin232; DOI: 10.61927/igmin232; Available at: igmin.link/p232
Copyright: © 2024 Rapp D This is an open access article distributed under the Creative Commons Attribution License, which permits unrestricted use, distribution, and reproduction in any medium, provided the original work is properly cited.
Rapp D. Human Missions to Mars. 3rd ed. Heidelberg, Germany: Springer-Praxis Books; Springer.
Genta G. Next Stop Mars. Heidelberg, Germany: Springer-Praxis Books; Springer; 2016.
Jones HW. The recent large reduction in space launch cost. In: 48th International Conference on Environmental Systems; 2018 Jul 8-12; Albuquerque, NM. Paper ICES-2018-81.
Drake BG. Mars design reference architecture 5.0 study - executive summary. 2008. Available from: https://www.nasa.gov/wp-content/uploads/2015/09/373669main_2008-12-04_mars_dra5_executive_summary-presentation.pdf?emrc=db5841
Jones HW. Take material to space or make it there? In: 2023 ASCEND Conference. Las Vegas, NV. 2023.
Space Ambition. The Starship: can one rocket change the entire space industry? 2024. Available from: https://spaceambition.substack.com/p/the-starship-can-one-rocket-change
Weinzieryl MC, Lucas K, Sarang M. Space X, economies of scale, and a revolution in space access. Harvard Business School Report 9-720-027; 2021.
Ash RL, Dowler WL, Varsi G. Feasibility of rocket propellant production on Mars. Acta Astronautica. 1978;5:705-724.
Sanders G, Kleinhenz J. In situ resource utilization (ISRU) "Envisioned future priorities". In: Space Resources Roundtable (SRR). Golden, CO. 2022.
Rapp D. Use of extraterrestrial resources for human space missions to Moon or Mars. 2nd ed. Heidelberg, Germany. Springer-Praxis Books; Springer. 2018.
Hanford AJ. Advanced life support research and technology. NASA Report NASA/CR-2004-208944C. 2004.
Hanford AJ. Advance life support baseline values and assumptions. NASA Report NASA/CR-2004-208941. 2006.
Allen CS, Burnett R, Charles J, et al. Guidelines and capabilities for designing human missions. NASA Report NASA/TM-2003-210785. 2003.
Jones H. Minimum risk space habitat and life support. In: 2020 International Conference on Environmental Systems. 2020; Paper ICES-2020-222.
Rapp D, Inglezakis V. Mars in situ resource utilization (ISRU) – a historical review and appraisal. Appl Sci. 2024;14:653.
Rapp D. Near term NASA Mars and lunar in situ propellant production (ISPP): complexity vs. simplicity. Space Sci Technol. 2024.
Bagdigian RM, et al. International space station environmental control and life support system mass and crewtime utilization in comparison to a long duration human space exploration mission. In: 45th International Conference on Environmental Systems. Seattle, WA. 2015; Paper ICES-2015-094.
Jones HW. Redundancy: how many unreliable spares are needed for high reliability and confidence on a time-limited mission? Personal communication; 2021.
Owens AC, et al. Integrated trajectory, habitat, and logistics analysis and trade study for human Mars missions. In: ASCEND 2020. Virtual. 2020.
Broyan JL, et al. NASA environmental control and life support technology development for exploration: 2020 to 2021 overview. In: 50th International Conference on Environmental Systems. 2021; Paper ICES-2021-384.
Owens AC, Cirillo WM, Piontek N, et al. Analysis and optimization of test plans for advanced exploration systems reliability and supportability. In: 50th International Conference on Environmental Systems; 2021; Paper ICES-2020-199.
Sanders G, Kleinhenz J, Linne D. NASA plans for in situ resource utilization (ISRU) development, demonstration, and implementation. Presentation to COSPAR. 2022. Available from: https://ntrs.nasa.gov/api/citations/20220008799/downloads/NASA%20ISRU%20Plans_Sanders_COSPAR-Final.pdf
Jones HW. Life support with failures and variable supply. In: 40th International Conference on Environmental Systems. Barcelona, Spain. 2010.
Using space-based resources for deep space exploration. 2024. Available from: https://www.nasa.gov/overview-in-situ-resource-utilization/
Elliott J, Sherwood B, Austin A, et al. ISRU in support of an architecture for a self-sustained lunar base. In: 70th International Astronautical Congress (IAC); Washington, DC. 2019. Paper IAC-19-D3,2A,2,x51412.
Zubrin R. Op-ed | Lunar Gateway or Moon Direct? Space News. 2019. Available from: https://spacenews.com/op-ed-lunar-gateway-or-moon-direct/